Transition from isotropic positive to negative thermal expansion by local Zr6O8 node distortion in MOF-801
Abstract
The chemical designability and diversity of metal-organic frameworks (MOFs) endow them with plenty of anomalous properties, such as negative thermal expansion (NTE). Herein, we investigated the thermal expansion behaviors of the well-known MOF-801, which has been widely used in water adsorption and gas separation. The analyses of variable temperature powder X-ray diffraction and Rietveld refinements revealed a fascinating transition from positive thermal expansion to NTE. Further in situ Raman spectra and pair distribution function investigations shed light on the transition being attributed to the local Zr6O8 node distortion rather than a long-range phase transition. Our findings will enhance the comprehension of NTE and contribute to the effective utilization of MOF-801 over a broad temperature range.
Keywords
INTRODUCTION
Thermal expansion, as an inherent physical property, significantly influences the performance and lifetime of materials. Consequently, designing smart materials with tunable or switchable thermal expansion properties is a significant yet challenging pursuit for materials scientists. The emergence of negative thermal expansion (NTE) materials presents a promising prospect[1,2]. Generally, the coefficients of thermal expansion (CTEs) can be modified by precisely controlling the chemical substitution[3]. For instance, substituting Sc3+ with isovalent but smaller radius cations such as Ga3+ or Fe3+ can induce local distortions, resulting in a continuous transition from NTE to positive thermal expansion (PTE)[4]. In the
Metal-organic frameworks (MOFs), a class of innovative porous materials constructed from inorganic metal nodes and organic linkers, have recently been recognized as an ideal platform for studying NTE[7-9]. The structural design flexibility and ease of decoration characteristics are expected to expand the range of NTE materials, offering additional strategies for tailoring CTEs[10]. To date, several MOFs have reported to exhibit NTE, such as IRMOF-1[11], HKUST-1[12], UiO-66(Hf)[13], MIL-68(In)[14], NU-1000(Zr)[15], Cd_sq[16], Cd(trz)Cl[17], and so on. A common characteristic of these MOFs is their construction using units with high symmetry, such as linear, triangular or square configurations. This structural feature imparts the framework with significant quantities of low-frequency phonons[18,19]. Upon heating, the transverse vibration, rotation or flipping tends to drag the connected nodes closer, resulting in the manifestation of NTE[20]. The close relationship between the ligand vibration and NTE suggests that introducing guest solvents or gases can effectively tailor NTE due to the steric hindrance effect[21-23]. Additionally, steric hindrance groups can be directly decorated as side groups of the ligands[24,25]. In addition to decorating organic ligands, the inorganic nodes, serving as pivotal elements within the framework, have been suggested to play a crucial role in determining lattice vibration behaviors. For example, the spin state of hexanuclear FeIII4FeII2 significantly influences the geometric configuration of the framework and the spin crossover can induce a colossal thermal expansion[26]. Recently, Chen et al. and Platero-Prats et al. discovered that the 8-connected Zr6O8 nodes can undergo local distortion upon heating, which can also contribute to the NTE[15,27].
In this research, we chose to investigate the thermal expansion property of MOF-801, which is composed of “zig-zag” type dicarboxylate ligands and Zr6O8 nodes[28]. Considering the widespread applications of the MOF-801 in gas separation and water adsorption[29-32], a detailed understanding of how the lattice and structure change upon heating (and cooling) will be useful for modifying its actual performance.
MATERIALS AND METHODS
MOF-801 samples were prepared and activated according to synthesis procedures as reported[28,33,34]. A mixture of ZrOCl2·8H2O (0.23 g, 0.70 mmol) and fumaric acid (0.081 g, 0.70 mmol) was dissolved in N,N-Dimethylformamide (DMF) within a 50-mL screw-capped glass jar, and 5.3 mL formic acid was added into the solution; then, the mixture was heated at 393 K for 24 h. The powder product was washed at least twice using water and alcohol and then dried at 383 K to obtain a white powder for subsequent experiment measurements. Prior to variable temperature X-ray powder diffraction (XRD), the powder sample was in situ dried at 383 K for 2 h to completely remove the adsorbed water molecules. Temperature-dependent
RESULTS AND DISCUSSION
MOF-801, Zr6O8(fumarate)6, is constructed by Zr6O8 nodes and fumaric acid ligands [Figure 1A-C]. The Zr6O8 node contains six zirconium atoms with μ3-O moieties coordinating at each face. The metal coordination is further completed by 12 carboxylate donor groups, which bridge the metal atoms along the octahedral edges. Furthermore, these 12-connected metal clusters are connected by fumarate ligands to form a three-dimensional cubic lattice with the fcu (face-centered cubic) topology (P n-3, a = 17.83480 Å). Structure arrangement in MOF-801 bears a resemblance to archetypal members of the extensively studied iso-reticular series of “UiO” MOFs, which also feature the Zr6O8 node but use different dicarboxylate ligands[37]. Considering the broad temperature range of MOF-801 potential applications, there is considerable interest in understanding its thermal expansion characteristic.
Figure 1. (A) Illustration of the crystal structure of MOF-801 viewed along the c-axis. (B) The structure of 12-connected Zr6O8 node in MOF-801 (Zr: blue, O: orange, C: grey, H: light green). (C) Chemical structure of the fumaric acid ligand.
To investigate the thermal expansion behavior of MOF-801, in situ variable temperature X-ray diffraction analyses were used [Supplementary Figure 1]. As shown in Figure 2A, upon heating from 100 to 500 K, an obvious peak shift (particularly in the high temperature range) toward high angles was observed, inferring lattice contraction according to the Bragg equation. Subsequently, when cooling from 500 to 100 K, a peak shift toward a low angle value can also be observed. To precisely reveal the lattice changes, Rietveld refinements were performed and the results are shown in Figure 2B and Supplementary Figure 2. From 100 to 375 K, the lattice parameter a monotonously expands with the coefficient αa = +4.30(7) MK-1. The contradictory result between the Rietveld refinements and observed peak shifts is due to the height of sample stage changes. Evidently, the thermal expansion behavior is different from previously reported Zr6O8-based analogs. Those MOFs constructed from linear dicarboxylates, such as 1,4-benzenedicarboxylic acid and 4,4′-(ethyne-1,2-diyl)dibenzoic acid, tend to show monotonous NTE owing to the transverse vibration of ligands[13,38], while, here, we found that the MOF-801 shows normal PTE. This may be caused by the unique “zig-zag” configuration of the fumarate ligand that can frustrate the correlated vibrations. Noticeably, in the high temperature range (375-500 K), a dramatically contracts with the coefficient
Figure 2. (A) The zoomed temperature-dependent X-ray diffraction profiles of MOF-801. (B) The unit cell parameters of MOF-801 with temperatures. Color code: first cycle, red; second cycle, blue. The symbols corresponding to the heating and cooling processes are shown as filled and empty scatters, respectively.
For framework materials, the NTE is generally believed to be associated with low frequency phonons[18,39,40]. To gain a deeper insight into the vibration modes of MOF-801, in situ variable temperature Raman spectroscopy investigation was conducted across a temperature range of 125-475 K with 25 K internals [Figure 3A]. Throughout the entire temperature range, no noticeable blue shift was observed for the low frequency bands (< 200 cm-1, Figure 3B). The high frequency vibration bands corresponding to the carboxylate stretching νas(COO), νs(COO) and -CH2 wagging also show similar trends
Figure 3. (A) In situ variable temperature Raman spectra of MOF-801. (B) Representative low-frequency Raman band shifts of MOF-801 vs. temperature.
Both the in situ powder X-ray diffraction and Raman spectroscopy indicated the absence of a phase transition in the long-range of the structure. With the concern of how the local structure changes, we performed PDF analysis based on the high-resolution X-ray total scattering. The PDF patterns predominantly feature atom-atom correlations, primarily focusing on the strongly scattering Zr atoms [Figure 4A]. This includes the Zr-O bond at distance of ~2.2 Å (path 1, Figure 4B), the Zr···Zr distances as the edges of the Zr6 octahedron at distance of ~3.5 Å (path 2) and as the two diagonal sites at distance of ~5.0 Å (path 3). The observed single Zr···O distances involve contributions from overlapping Zr-carboxylate and Zr-oxo distances. The singular Zr···Zr distance at 3.5 Å corresponds to the 12 equivalent Zr···Zr distances within the regular octahedron, highlighting the structural intricacies of MOF-801 at the local level. Before 375 K, no obvious peak changes, including peak intensity and position, can be observed, while a noticeable alteration in the local structure becomes evident when the temperature exceeds 375 K. As shown in Figure 4C, the initially singular peak at ~3.5 Å that corresponds to the Zr···Zr pair splits into two peaks at 3.3 and 3.8 Å with an intensity ratio of nearly 2:1. The peak at ~5 Å also broadens, indicating a wider distribution of Zr···Zr distances and disorder. These changes of Zr···Zr pairs suggest that the originally regular Zr6 octahedron become distorted Figure 4D, consistent with a recently reported 8-connected Zr6O8 node in NU-1000 that capped with H2O or OH[15]. As discussed by Chen et al., this type of distortion behavior possesses relatively lower reversibility, which may be the origin of difference between the first and second cycling measurements[15]. To quantify the distortion extent in the local structure, the change in the crucial Zr···O distance was evaluated. As shown in Supplementary Figure 4, the change of Zr···O distance aligns with the trend observed in the CTEs depicted in Figure 2B. So, we have reason to believe that the distorted Zr6O8 nodes possess a smaller volume, giving rise to observed volume contraction at high temperatures.
Figure 4. (A) PDF patterns of MOF-801 at 300 K (path 1: Zr-O atom pair, path 2: Zr···Zr atom pair, path 3: Zr···Zr atom pair). (B) Schematic diagram of the Zr6O8 node, showing paths 1, 2 and 3, respectively. (C) Temperature-dependent PDF patterns in the range of 1.5-5.5 Å. (D) Illustration of the local Zr6O8 node distortion with temperature.
CONCLUSIONS
In summary, we investigated the thermal expansion behavior of MOF-801, a significant representative of typical Zr-based MOFs. In the low temperature range (< 375 K), it exhibits normal PTE due to the transverse vibration of dicarboxylate ligands being frustrated by the “zig-zag” configuration of fumarate ligands. Intriguingly, a distinctive transition from PTE to NTE was identified in the temperature range of 375~500 K. Detailed analyses through in situ powder X-ray diffraction and Raman spectroscopy analysis excluded the occurrence of long-range phase transitions. The PDF study unveiled a temperature-induced local distortion of the Zr6O8 node, contributing to the occurrence of NTE. This sheds light on the dynamics of the local structure within a specific temperature range, enhancing our understanding of NTE. The current observation of NTE in MOF-801 holds promise for exploring diverse potential applications.
DECLARATIONS
Authors’ contributions
Conceived and designed the study: Xing X
Prepared the samples and collected the data: Ma R
Analyzed data and wrote the main draft of the paper: Ma R, Liu Z, Xing X
Examined the thermal expansion results: Chen L, Li Q, Lin K, Chen X, Deng J
Conducted the Synchrotron radiation measurements: Ohara K, Li Q
All authors discussed the results and commented on the manuscript.
Availability of data and materials
Not applicable.
Financial support and sponsorship
This research was supported by the National Key R&D Program of China (2020YFA0406202), National Natural Science Foundation of China (22090042, 22005340 and 22175018), and Guangxi BaGui Scholars Special Funding (2019M660446). The variable-temperature X-ray total-scattering Measurements were performed with the approval of Spring-8 (proposal number 2021A1060).
Conflicts of interest
All authors declared that there are no conflicts of interest.
Ethical approval and consent to participate
Not applicable.
Consent for publication
Not applicable.
Copyright
© The Author(s) 2024.
Supplementary Materials
REFERENCES
1. Li Q, Lin K, Liu Z, et al. Chemical diversity for tailoring negative thermal expansion. Chem Rev 2022;122:8438-86.
2. Takenaka K. Negative thermal expansion materials: technological key for control of thermal expansion. Sci Technol Adv Mater 2012;13:013001.
3. Chen J, Hu L, Deng J, Xing X. Negative thermal expansion in functional materials: controllable thermal expansion by chemical modifications. Chem Soc Rev 2015;44:3522-67.
4. Hu L, Chen J, Fan L, et al. Zero thermal expansion and ferromagnetism in cubic Sc1-xMxF3 (M = Ga, Fe) over a wide temperature range. J Am Chem Soc 2014;136:13566-9.
5. Song Y, Sun Q, Xu M, et al. Negative thermal expansion in (Sc,Ti)Fe2 induced by an unconventional magnetovolume effect. Mater Horiz 2020;7:275-81.
6. Zhou H, Tao K, Chen B, et al. Low-melting metal bonded MM’X/In composite with largely enhanced mechanical property and anisotropic negative thermal expansion. Acta Mater 2022;229:117830.
7. Balestra SR, Bueno-Perez R, Hamad S, Dubbeldam D, Ruiz-Salvador AR, Calero S. Controlling thermal expansion: a metal-organic frameworks route. Chem Mater 2016;28:8296-304.
8. Zhang JP, Zhou HL, Zhou DD, Liao PQ, Chen XM. Controlling flexibility of metal-organic frameworks. Nat Sci Rev 2018;5:907-19.
9. Burtch NC, Baxter SJ, Heinen J, et al. Negative thermal expansion design strategies in a diverse series of metal-organic frameworks. Adv Funct Mater 2019;29:1904669.
10. Schneider C, Bodesheim D, Ehrenreich MG, et al. Tuning the negative thermal expansion behavior of the metal-organic framework Cu3BTC2 by retrofitting. J Am Chem Soc 2019;141:10504-9.
11. Dubbeldam D, Walton KS, Ellis DE, Snurr RQ. Exceptional negative thermal expansion in isoreticular metal-organic frameworks. Angew Chem Int Ed 2007;46:4496-9.
12. Wu Y, Kobayashi A, Halder GJ, et al. Negative thermal expansion in the metal-organic framework material Cu3(1,3,5-benzenetricarboxylate)2. Angew Chem Int Ed 2008;47:8929-32.
13. Cliffe MJ, Hill JA, Murray CA, Coudert FX, Goodwin AL. Defect-dependent colossal negative thermal expansion in UiO-66(Hf) metal-organic framework. Phys Chem Chem Phys 2015;17:11586-92.
14. Liu Z, Li Q, Zhu H, et al. 3D negative thermal expansion in orthorhombic MIL-68(In). Chem Commun 2018;54:5712-5.
15. Chen Z, Stroscio GD, Liu J, et al. Node distortion as a tunable mechanism for negative thermal expansion in metal-organic frameworks. J Am Chem Soc 2023;145:268-76.
16. Liu Z, Ma R, Deng J, Chen J, Xing X. Molecular packing-dependent thermal expansion behaviors in metal squarate frameworks. Chem Mater 2020;32:2893-8.
17. Liu Z, Fan L, Xing C, Wang Z. Negative thermal expansion in the noncarboxylate based metal-organic framework Cd(trz)Cl. ACS Mater Lett 2023;5:1911-5.
18. Zhou W, Wu H, Yildirim T, Simpson JR, Walker ARH. Origin of the exceptional negative thermal expansion in metal-organic framework-5 Zn4O(1,4-benzenedicarboxylate)3. Phys Rev B 2008;78:054114.
19. Rimmer LH, Dove MT, Goodwin AL, Palmer DC. Acoustic phonons and negative thermal expansion in MOF-5. Phys Chem Chem Phys 2014;16:21144-52.
20. Lock N, Wu Y, Christensen M, et al. Elucidating negative thermal expansion in MOF-5. J Phys Chem C 2010;114:16181-6.
21. Goodwin AL, Chapman KW, Kepert CJ. Guest-dependent negative thermal expansion in nanoporous prussian blue analogues
22. Zhou HL, Zhang YB, Zhang JP, Chen XM. Supramolecular-jack-like guest in ultramicroporous crystal for exceptional thermal expansion behaviour. Nat Commun 2015;6:6917.
23. Auckett JE, Barkhordarian AA, Ogilvie SH, et al. Continuous negative-to-positive tuning of thermal expansion achieved by controlled gas sorption in porous coordination frameworks. Nat Commun 2018;9:4873.
24. Baxter SJ, Schneemann A, Ready AD, Wijeratne P, Wilkinson AP, Burtch NC. Tuning thermal expansion in metal-organic frameworks using a mixed linker solid solution approach. J Am Chem Soc 2019;141:12849-54.
25. Henke S, Schneemann A, Fischer RA. Massive anisotropic thermal expansion and thermo‐responsive breathing in metal-organic frameworks modulated by linker functionalization. Adv Funct Mater 2013;23:5990-6.
26. Sun HY, Meng YS, Zhao L, et al. Colossal anisotropic thermal expansion through coupling spin crossover and rhombus deformation in a hexanuclear {FeIII4FeII2} Compound. Angew Chem Int Ed 2023;62:e202302815.
27. Platero-Prats AE, Mavrandonakis A, Gallington LC, et al. Structural transitions of the metal-oxide nodes within metal-organic frameworks: on the local structures of NU-1000 and UiO-66. J Am Chem Soc 2016;138:4178-85.
28. Furukawa H, Gándara F, Zhang YB, et al. Water adsorption in porous metal-organic frameworks and related materials. J Am Chem Soc 2014;136:4369-81.
29. Kim H, Rao SR, Kapustin EA, et al. Adsorption-based atmospheric water harvesting device for arid climates. Nat Commun 2018;9:1191.
31. Xu W, Yaghi OM. Metal-organic frameworks for water harvesting from air, anywhere, anytime. ACS Cent Sci 2020;6:1348-54.
32. Iacomi P, Formalik F, Marreiros J, et al. Role of structural defects in the adsorption and separation of C3 hydrocarbons in Zr-fumarate-MOF (MOF-801). Chem Mater 2019;31:8413-23.
33. Dai S, Nouar F, Zhang S, Tissot A, Serre C. One-step room-temperature synthesis of metalIV carboxylate metal-organic frameworks. Angew Chem Int Ed 2021;60:4282-8.
34. Li CN, Wang SM, Tao ZP, et al. Green synthesis of MOF-801(Zr/Ce/Hf) for CO2/N2 and CO2/CH4 separation. Inorg Chem 2023;62:7853-60.
36. Yang X, Juh’as P, Farrow CL, Billinge SJL. xPDFsuite: an end-to-end software solution for high throughput pair distribution function transformation, visualization and analysis. 2015. Available from: https://arxiv.org/abs/1402.3163 [Last accessed on 12 Apr 2024].
37. Cavka JH, Jakobsen S, Olsbye U, et al. A new zirconium inorganic building brick forming metal organic frameworks with exceptional stability. J Am Chem Soc 2008;130:13850-1.
38. Zhou HL, Bai J, Ye JW, et al. Thermal and gas dual-responsive behaviors of an expanded UiO-66-type porous coordination polymer. Chempluschem 2016;81:817-21.
39. Zhang X, Jiang X, Molokeev MS, Wang N, Liu Y, Lin Z. Two-dimensional negative thermal expansion in a crystal of LiBO2. Chem Mater 2022;34:4195-201.
40. Liu Z, Jiang X, Wang C, et al. Near-zero thermal expansion coordinated with geometric flexibility and π-π interaction in anisotropic [Zn8(SiO4)(m-BDC)6]n. Inorg Chem Front 2019;6:1675-9.
Cite This Article
Export citation file: BibTeX | RIS
OAE Style
Ma R, Liu Z, Chen L, Li Q, Lin K, Chen X, Deng J, Ohara K, Xing X. Transition from isotropic positive to negative thermal expansion by local Zr6O8 node distortion in MOF-801. Microstructures 2024;4:2024023. http://dx.doi.org/10.20517/microstructures.2023.70
AMA Style
Ma R, Liu Z, Chen L, Li Q, Lin K, Chen X, Deng J, Ohara K, Xing X. Transition from isotropic positive to negative thermal expansion by local Zr6O8 node distortion in MOF-801. Microstructures. 2024; 4(2): 2024023. http://dx.doi.org/10.20517/microstructures.2023.70
Chicago/Turabian Style
Ma, Rui, Zhanning Liu, Liang Chen, Qiang Li, Kun Lin, Xin Chen, Jinxia Deng, Koji Ohara, Xianran Xing. 2024. "Transition from isotropic positive to negative thermal expansion by local Zr6O8 node distortion in MOF-801" Microstructures. 4, no.2: 2024023. http://dx.doi.org/10.20517/microstructures.2023.70
ACS Style
Ma, R.; Liu Z.; Chen L.; Li Q.; Lin K.; Chen X.; Deng J.; Ohara K.; Xing X. Transition from isotropic positive to negative thermal expansion by local Zr6O8 node distortion in MOF-801. Microstructures. 2024, 4, 2024023. http://dx.doi.org/10.20517/microstructures.2023.70
About This Article
Special Issue
Copyright
Data & Comments
Data
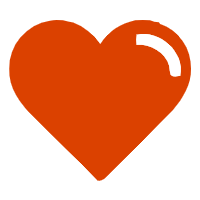

Comments
Comments must be written in English. Spam, offensive content, impersonation, and private information will not be permitted. If any comment is reported and identified as inappropriate content by OAE staff, the comment will be removed without notice. If you have any queries or need any help, please contact us at support@oaepublish.com.