A theoretical review of passivation technologies in perovskite solar cells
Abstract
Perovskite solar cells have demonstrated remarkable progress in recent years. However, their widespread commercialization faces challenges arising from defects and environmental vulnerabilities, leading to limitations in energy conversion efficiency and device stability. To overcome these hurdles, passivation technologies have emerged as a promising avenue. These passivators are strategically applied at the interface between perovskite absorbers and charge transport layers to mitigate the adverse effects of defects and environmental factors. While prior reviews have predominantly focused on experimental observations, a comprehensive theoretical understanding of the passivators has been lacking. This review focuses on recent advancements in first-principles density functional theory studies that delve into the fundamental properties of passivators and their intricate interactions with perovskite materials and charge transport layers. By exploring the atomic-level roles of passivators, this review elucidates their impact on critical parameters such as open circuit voltage (Voc), short circuit current density (Jsc), fill factor, and the overall stability of perovskite solar cells. The synthesis of theoretical insights from these studies can serve as guidelines for the molecular design of passivators with the ultimate objective of advancing the commercialization of high-performance perovskite solar cells.
Keywords
INTRODUCTION
Over the last decade, remarkable strides have been achieved in the field of perovskite solar cells (PSCs), establishing them as exceptionally promising contenders for widespread commercialization[1]. The distinctive optoelectronic characteristics of metal-halide perovskites, encompassing a high absorption coefficient, extended carrier diffusion lengths (L), and adaptable bandgaps, contribute to their remarkable efficacy in capturing and converting sunlight into electricity[1-3]. Additionally, using solution-based deposition methods for perovskite film formation presents the potential for large-scale, cost-effective manufacturing processes, underscoring PSCs as a promising technology for achieving widespread adoption of renewable energy[4,5]. To date, PSCs have achieved impressive power conversion efficiencies (PCEs) exceeding 25% in both normal n-i-p and inverted p-i-n structures in laboratory settings, rapidly approaching the theoretical Shockley-Queisser efficiency limit[3]. Nonetheless, some challenges still need to be addressed before their commercial adoption.
A significant hindrance is the inherent formation of defect-trapping states during the fabrication of PSCs, with these defects exerting a pronounced negative impact on overall device performance and resulting in diminished PCEs[6-8]. Additionally, the degradation of perovskite light absorbers under diverse environmental factors, such as moisture, poses another significant challenge for PSC commercialization[9,10]. The susceptibility of perovskite materials to these external factors can lead to decreased efficiency over time, limiting their practical applicability. To position PSCs as a highly competitive and sustainable technology for solar energy conversion, their long-term stability (LTS) and reliability must be meticulously addressed to ensure consistent performance over extended operational periods.
To address the challenges associated with defect formation and light absorber degradation during PSC operation, substantial efforts have been devoted to developing passivation techniques[11]. These techniques aim to either suppress the formation of defects or enhance the stability of the light absorbers, thereby improving the overall performance of PSCs. Passivators are commonly introduced at the interface between the perovskite light absorbers and the charge transport layers, including the electron transport layer (ETL) and the hole transport layer (HTL) [Figure 1A]. Strategically incorporating passivators into the device architecture can reduce the defect density within the perovskite layer or between interfaces, significantly enhancing carrier recombination dynamics and charge extraction efficiency. Consequently, the key parameters related to the energy conversion performance of PSCs, including open-circuit voltage (VOC), short-circuit current density (JSC), and fill factor (FF), can be substantially improved. Determination of these parameters involves measuring and analyzing the current-voltage (J-V) characteristics of the device [Figure 1B]. Furthermore, passivation techniques exhibit promise in extending the lifespan of perovskite light absorbers by mitigating the detrimental effects of environmental factors, such as moisture, thereby preserving the structural integrity and optoelectronic properties of perovskite materials. This enhanced stability is crucial for the long-term performance and durability of PSCs, facilitating their practical implementation in real-world applications.
Figure 1. (A) Illustration of the structure of perovskite solar cells, including ETL, perovskite light absorbers and HTL. The passivation layer is introduced to improve the energy conversion parameters. Three important parameters, including VOC, JSC, and FF, are shown in (B) the J-V curve.
Various passivators and passivation technologies have been explored in the realm of PSCs. Potential passivators encompass cation and anions dopants[12], one- (1D)[13] or two-dimensional (2D) materials[14], Lewis acid-based adducts[15], alkylammonium halogen salts[16], and hydrophobic organic molecules[17]. Several comprehensive review papers have delved into the properties and potential applications of these passivation materials[6,18,19]. However, the full atomic-level impact of passivators on solar cell performance remains challenging to grasp solely through experimental results, owing to the limitations of characterization techniques. In this regard, theoretical investigations offer a valuable supplement to experimental studies, offering the potential to unveil the structure-function-performance relationship of passivators. Surprisingly, the theoretical understanding of the function of passivators in PSCs has received a scant review, primarily because most theoretical studies have been integrated into literature employing a combined experimental and theoretical approach. Relatively few independent theoretical studies on this topic have been conducted and systematically summarized.
To fill this knowledge gap, we consolidate existing theoretical knowledge to facilitate the translation of theoretical insights into practical applications. In this review, we present an overview of computational methods used in theoretical studies on passivators and passivation techniques in PSCs. Recent examples are utilized to elucidate the theoretical understanding of passivation in enhancing energy conversion efficiency, including improvements in VOC, JSC, and FF. This review also addresses the role of passivators in prolonging the lifetime of PSCs. Drawing on recent theoretical advances, selection criteria are then proposed for designing PSC passivators.
METHODOLOGIES
Density functional theory
Density functional theory (DFT) is a widely employed method for investigating the structure-function-performance relationship of passivators in PSCs due to its computational efficiency and acceptable accuracy[20-26]. In DFT, the exact form of exchange-correlation (XC) functional is unknown, necessitating approximations. A common DFT approach involves using semi-local XC functionals with the generalized gradient approximation (GGA)[13,14]. However, conventional DFT methods at the GGA level often fall short of accurately capturing van der Waals (vdW) forces. Prior research highlights the critical role of precise vdW force treatment in describing the structural properties of PSC materials, particularly in the presence of heavy elements such as Pb and I[27-29]. Therefore, incorporating vdW correction in the DFT method becomes essential.
Furthermore, DFT at the GGA level tends to underestimate the bandgap of semiconductors[30-33]. While GGA-level calculations can replicate the bandgap of certain organic-lead perovskite materials, their band structures become inaccurate without accounting for spin-orbit coupling (SOC) effects[34-37]. SOC arises from the interaction between the magnetic dipole of an electron and the electric field generated by atomic nuclei, becoming prominent in heavy atoms due to relativistic effects. For instance, SOC calculations on MAPbI3 reveal band splitting, resulting in a significantly smaller bandgap compared to experimental values[38,39]. Additionally, the positions of the conduction band minimum (CBM) and valence band maximum (VBM) are notably altered, affecting the optical properties and effective masses of charge carriers. Therefore, accurate evaluation of electronic and optical properties in theoretical studies necessitates the consideration of SOC effects[38,40-63]. After considering the SOC, the calculated bandgap of MAPbI3 is significantly smaller than the experimental value. To produce more accurate electronic properties, a hybrid functional including a fraction of exact non-local Hartree-Fock (HF) exchange was introduced[12]. To this end, the accurate electronic and optical properties of lead (Pb)-based perovskite light absorbers can only be achieved using the hybrid DFT method with the consideration of the SOC effect. It should be noted that hybrid DFT calculations considering the SOC effect are computationally intense, and most theoretical studies on passivation techniques tend to neglect this effect due to its minimal influence on structural and energetic properties[64].
Theoretical parameters related to VOC
The VOC refers to the voltage generated by a solar cell in the absence of current flow due to a broken circuit or exceptionally high resistance. Theoretically, it corresponds to the energy difference between the CBM and VBM levels when considering only the light absorbers in the solar cell. In solar cell devices, ETLs and HTLs are utilized to facilitate the separation and efficient transfer of photo-generated electrons (e-) and holes (h+), reducing their recombination. Typically, when an electron is photo-excited from the valence band (VB) to the conduction band (CB), photoelectrons transfer from the CB of the absorber to the CB of the ETL, while photo-holes migrate from the VB of the absorber to the VB of the HTL. To enable the effective transfer of photoelectrons from the light absorber to the ETL, the CBM of the ETL should be lower than that of the perovskite materials. Similarly, the VBM of the HTL should be higher than that of the light absorber, guiding the movement of photo-holes from the absorber to the HTL. Consequently, the theoretical VOC value of the device becomes the difference between the CBM of the ETL and the VBM of the HTL.
The CBM and VBM levels can be theoretically determined through the DFT calculation. The CBM and VBM locations can be calculated with respect to the vacuum energy level (Evac). The Evac can be calculated by averaging the electrostatic potential along the normal direction of the surface. For metallic materials, there are no CBM and VBM. Instead, the work function (Φ) is used to represent the highest occupied energy level of the surface. The band structure analysis can further identify the CBM and VBM associated with k-vectors. In direct semiconductors, CBM and VBM share the same k-vector, while in indirect band gap materials, their k-vectors are different. It is worth noting that band structure calculations at the DFT-GGA level can result in severe underestimations of the CBM, leading to a significant reduction of 30% to 40% in the overall bandgap[30-33]. To address this, hybrid DFT methods incorporating HF exchange have been shown to provide more accurate theoretical bandgap, CBM and VBM results[65].
Theoretical parameters related to JSC
The JSC represents the maximum current density achievable by a cell when operating under negligible resistance and zero voltage. In the context of solar cells, the electrical conductivity of the materials used, such as the passivation layer, can influence the JSC value. Higher electrical conductivity allows for better charge transport, which results in higher current densities under short-circuit conditions. By understanding the relationship between the JSC and the electrical conductivity of passivation materials, researchers can make informed choices in material selection and device design to enhance the performance of solar cells. Therefore, optimizing the electrical conductivity of the materials within a solar cell can help maximize the
The Drude model provides a valuable tool for estimating and predicting electrical conductivity behaviors, thereby aiding in developing more efficient solar cell technologies. In this model, conductivity depends on parameters such as carrier concentration (n), charge (e), and mobility (m)[66]. The concentration of carriers is influenced by the density of defects and the inherent ability to generate carriers. The carrier mobility, on the other hand, is affected by the L of charge carriers. The L values are determined by the effective mass of the exciton (m*)[67]. The m* value can be theoretically calculated using
where
Previous studies underscore the necessity of considering the SOC effect for accurately predicting effective masses, particularly in materials with heavy elements[34]. The incorporation of SOC in the calculations increases the band dispersions, resulting in reduced effective masses for both electrons and holes. For example, Umari et al. investigated the effective masses of electrons and holes of MAPbI3 using different methods[68]. Without accounting for SOC, the average effective masses were 0.73 m0 for electrons and
The JSC of a solar cell also heavily depends on the optical properties of the device and is directly proportional to the power of the incident light. Therefore, the absorption and light-harvesting capabilities of the solar cell play a crucial role in determining JSC. Enhancing the optical properties, including enhanced light absorption and minimized reflection or transmission losses, can increase JSC. To this end, an effective passivator should exhibit low reflectivity and absorption, minimizing its impact on the light absorption by the perovskite materials. To assess this, the absorption coefficients (α(ω) × 105 cm-1) and optical reflectivity (R(ω)) can be calculated from complex dielectric function (ε(ω) = ε1(ω) + iε2(ω)) using
Theoretical parameters related to FF
The FF is utilized to determine the maximum power output of a solar cell. This maximum power point (PMP) corresponds to the ideal voltage (Vmp) and current density (Jmp) at which the solar cell operates most efficiently, maximizing its power generation (See Figure 1B). The FF can be improved by increasing PMP, which can be achieved by enhancing the charge collection efficiency[69]. The charge collection efficiency in solar cells is influenced by the charge transfer between different layers. The binding strength between specific layers within the solar cell structure plays a crucial role. A strong binding between the layers efficiently facilitates charge transfer (electrons and holes) across interfaces, minimizing charge recombination losses. In this regard, the charge collection efficiency can be improved by optimizing the binding strength between these layers, leading to enhanced overall performance of the solar cell.
The binding strength between molecular passivators can be evaluated based on their adsorption energy
where Ead, Esurf, and Ead/surf are the energies of the passivator, the clean perovskite surface, and the perovskite surface with passivators, respectively, and n is the number of passivators in each surface cell.
For the 2D materials, their binding strength (ΔEb) can be calculated by
where E2D and Ehybrid are the energies of 2D monolayer and their interface with 2D monolayers on perovskite surface, respectively. The A is the area of the interface within each unit cell.
Theoretical parameters related to stability
Mitigating the instability of halide perovskites under operational conditions is a crucial objective in the field of PSCs[70]. One of the primary challenges in achieving this stability lies in preventing the adsorption of water, which can degrade the perovskite material over time[71]. To address this issue, passivators act as protective layers to block the pathways for water adsorption. By introducing passivators that have a stronger affinity to the perovskite surface than water, their adsorption can outcompete that of water molecules. This competitive adsorption prevents the undesired interaction of water with the perovskite material and maintains its stability during operation.
To assess the performance of passivation, it is crucial to evaluate the effectiveness of the passivator compared to water adsorption. This evaluation can be conducted through theoretical investigations using DFT calculations. By employing theoretical investigations and assessing the competitive adsorption of passivators with water, researchers can gain insights into the potential of diverse passivators to protect halide perovskites from degradation under operational conditions. Therefore, theoretical calculations can provide valuable guidance in designing and selecting effective passivation strategies. The DFT at the GGA level often allows researchers to simulate and predict the adsorption behavior of molecules and materials at the atomic level[72]. By calculating the adsorption or binding energies using Eq. 4 and 5, the passivation performance of different passivator candidates can be quantitatively assessed. Since the negative adsorption energy values suggest the exothermic process, a lower adsorption energy indicates a stronger interaction between the passivator and the perovskite surface, suggesting a more effective passivation strategy.
PASSIVATION FOR IMPROVING VOC
The optimization of band alignment between the ETL/HTL and perovskite light absorbers is crucial for improving the VOC in PSCs. To achieve optimal band alignment, passivators need to possess energy levels that bridge the gap between the HTL/ETL and the perovskite absorbers. The ideal alignment of band edges for efficient photovoltaic applications is not always naturally achieved due to the inherent electronic properties of the materials. Misalignment between the charge transport layers and light absorbers can result in internal voltage drops (IVDs), reducing the theoretical VOC[73-78]. Thus, passivation strategies aim to modify the CBM and VBM positions, as illustrated in Figure 2A.
Figure 2. (A) Illustration of the role of the ideal passivator at the interface between ETL and perovskite materials or between HTL and perovskite materials to change the CBM or VBM locations, respectively to further improve the VOC. (B) The passivator Cs+ was used to fill the MA+ vacancy, leading to decreased VBM location of the perovskites and increased VOC[85]. Copyright 2022 Wiley. (C) The use of ACh+ passivators to fill the MA+ vacancy leads to removing the deep trap states, as evidenced by the DOS analysis[85]. Copyright 2022 Wiley. (D) The use of ACh+ passivators to fill the MA+ vacancy leads to removing the shallow trap states, as evidenced by the DOS analysis[87]. Copyright 2017 Wiley.
In recent studies, the effectiveness of passivators in improving the VOC of PSCs has been demonstrated through theoretical investigations and experimental validations. Various passivation approaches have been proposed and investigated in recent studies, including surface passivation[79], interface engineering[80], defect passivation[81,82], and composition optimization[83]. Each approach has specific challenges associated with band misalignment in PSCs. Theoretical investigations using DFT calculations provide valuable insights into the band structures of passivated layers within solar cells, allowing for the design and evaluation of effective passivation strategies.
Passivation at the adsorber-ETL interface
Che et al. systematically studied the effect of carbonyl, hydrazine and phenyl groups in different passivators using three kinds of hydrazide derivatives (benzoyl hydrazine (BH), formohydrazide (FH), and benzamide (BA))[84]. Their DFT calculations at the GGA level reveal that the carboxyl and hydrazine groups in BH can effectively interact with surface Pb2+, employing a synergistic double coordination approach. This interaction passivates both shallow- and deep-level defects by increasing the energy barrier for lattice distortion. The adsorption of BH passivators can lead to a noteworthy increase in the CBM energy level of CsPbI3, resulting in a corresponding increase in the VOC from 1.17 to 1.24 V. Zhang et al. investigated the role of acetylcholine (ACh+) on enhancing the VOC of PSCs[85]. Combining experimental and theoretical approaches, their DFT calculations at the Perdew-Burke-Ernzerhof (PBE)-GGA level focused on the electronic properties of the MAPbI3 (100) surface. Density of states (DOS) analyses unveiled the presence of Pb-I antisites and I vacancies, introducing deep and shallow trap states in the band gap, consequently diminishing the VOC. However, after the adsorption of ACh+ on the defective sites, both deep and shallow trap states were successfully removed or reduced. The results indicated that introducing ACh+ reduced the surface energies of the MA-I and Pb-I terminated surfaces, leading to the passivation of surface defect states. As a result, both the deep and shallow trap states can be efficiently removed, as evidenced by the DOS analysis [Figure 2B and C]. The DOS images further support the increase of the CBM after removing defect states, providing a rationale for the observed increase in VOC from 1.12 to 1.21 V after the passivation with ACh+.
Dong et al. also demonstrated that chlorobenzenesulfonic potassium salts can interact with uncoordinated tin ions in tin oxide ETL[86]. Calculations done with PBE-GGA and DFT-D3 revealed that the carbon-chlorine bonds in the salts filled tin oxide oxygen vacancies, improving band-level alignment. Consequently, the VOC shifted from 1.127 V toward an ideal value, 1.163 V. Furthermore, Batmunkh et al. employed passivators to enhance the VOC by tuning the CBM of the ETL in the n-i-p device[87]. In their study, the introduction of single-wall carbon nanotubes (SWCNTs) between TiO2 ETL and perovskite increased the VOC from 0.87 to 0.93 V. First-principles DFT calculations at the GGA-PBE level revealed that the CBM level of TiO2 ETL increased by 0.23 V upon SWCNT adsorption on the anatase TiO2 (101) surface, as shown in Figure 2D. This increased CBM location of the ETL aligns with the experimental observations, resulting in an enhanced theoretical VOC. Additionally, Chavan et al. explored passivation on the ETL to enhance the
Passivation at the adsorber-HTL interface
Jiang et al. reported that surface engineering by using 3-(amino methyl) pyridine (3-APy) as a passivator can efficiently reduce the perovskite surface roughness and surface potential fluctuations associated with the surface states[89]. Moreover, the negatively charged pyridine rings in the 3-Apy passivator can react with the surface formamidinium (FA) ions. DFT calculations at the GGA level reveal that this reaction facilitates the formation of surface iodine vacancies, enabling n-type doping. As a result, the VBM of the perovskite experienced a notable shift from 0.80 to 1.51 eV, explaining the enhanced VOC of 1.19 V in the experiments. Bati et al. used Cs-doped Ti3C2Tx MXene as a passivator in PSCs[90]. Their DFT calculations at the GGA level with an optB86b vdW correction method showed the formation of an MA+ cation vacancy in the MAPbI3 perovskite, which led to a shift in the VBM from -5.88 to -5.60 V to the Evac. However, the subsequent adsorption of Cs+ at the MA+ vacancy restored the VBM to -5.90 V. This shift in the VBM can be attributed to the strong adsorption of Cs+ with an adsorption energy of -5.13 eV, effectively eliminating defect states caused by the absence of MA+ on the surface. The introduction of Cs-doped Ti3C2Tx MXene resulted in an enhanced VOC from 1.05 to 1.10 V, highlighting the role of the VBM shift in providing device performance.
Zhang et al. also discovered a passivating material to increase the charge carrier lifetimes and improve band-level alignments[91]. Here, the PBE-GGA method was used to understand the electronic conditions. They used the electron-dense benzene ring of Boc-S-4-methoxy-benzyl-L-cysteine (BMBC) to strengthen interactions with undercoordinated lead ions. These mechanisms improved band edge alignments to increase the VOC from 1.144 to 1.249 V. On top of this, Zhang et al. have used trifluoroacetamidine for defect suppression, again with the PBE functional[92]. Here, hydrogen bonds from the fluorine groups interacted with the perovskite to reduce recombination. The reduction in recombination rates at the grain boundaries allowed the VOC to be brought up from 1.10 to 1.16 V.
PASSIVATION FOR IMPROVING JSC
In contrast to the extensive research on passivation for improving VOC, both experimental and theoretical investigations on the passivation effect on JSC are relatively rare. The JSC values are influenced by various factors, including the presence of defects and the optical properties of the solar cell. Defects can affect JSC by influencing the minority-carrier collection probability. Their presence can create recombination centers that reduce the efficiency of collecting minority carriers, leading to a decrease in JSC. To this end, various passivation strategies have been proposed to enhance light absorption, minimize recombination, and optimize charge carrier collection efficiency to increase JSC. Accordingly, the theoretical studies on passivation mainly focus on suppressing charge recombination rates.
Batmunkh et al. employed phosphorene (P) nanosheets as passivators to improve carrier mobilities in planar n-i-p PSCs[93]. Utilizing DFT calculations at the PBE-GGA level for initial atomic structure optimization and subsequent hybrid DFT calculations with the HSE06 functional for accurate electronic properties, the CBM position of 5-layer phosphorene was found to bridge between the CBM of TiO2 ETL and the perovskite. This band alignment indicated that phosphorene could act as a bridge between the ETL and the light absorber, facilitating the transfer of photo-excited electrons, reducing charge recombination rates, and increasing JSC [Figure 3A-C]. Experimental results showed a modest increase in JSC from 22.21 to 23.32 mA/cm2 after introducing phosphorene passivators. The effectiveness of using black phosphorene as a passivator to improve JSC has also been experimentally confirmed by Macdonald et al.[94].
Figure 3. (A) The atomic structure and (B) density of states of five-layer phosphorene, shifted with respect to the vacuum level EV. The vertical purple lines indicate the positions of the CBM and VBM. (C) Energy diagram of five-layer phosphorene incorporated TiO2 photoelectrodes-based PSC[93]. Copyright 2019 Wiley. (D) Band structures and TDOS of phosphorene (P), arsenene (As), antimonene (Sb), and bismuthine (Bi) with α-phase and β-phase. The dashed lines indicate the direction for calculating the effective masses of electrons and holes[95]. Copyright 2023 American Chemical Society. (E) Simulated reflectivity (R(ω)) spectra of phosphorene (P), arsenene (As), antimonene (Sb), and bismuthine (Bi) with the α-phase and β-phase[95]. Copyright 2023 American Chemical Society. (F) Calculated absorption coefficients (α(ω) × 105 cm-1) of phosphorene (P), arsenene (As), antimonene (Sb), and bismuthine (Bi) with the α-phase and β-phase[95]. Copyright 2023 American Chemical Society.
Inspired by the experimental results, Allen et al. further conducted DFT calculations at the hybrid HSE06 level to investigate the effective mass of various Group VA monolayers, including black phosphorene from their band structures [Figure 3D][95]. The results showed that Group VA monolayers have small effective masses of charge carriers, indicating promise for their use as passivators for both ETL and HTL interfaces. Notably, Cheng et al. predicted high hole mobility for 2D β-antimony, and HSE06 results indicated that all
PASSIVATION For IMPROVING FF
The FF is a critical parameter that significantly influences the overall efficiency of a solar cell. It represents the extent to which the actual power output of the cell approaches the maximum theoretical power. Therefore, improving the FF is essential to enhance the overall performance of the solar cell. One effective strategy to boost the FF is to increase the anchoring strength between the various components of the cell. Reinforcing the interlayer interactions and bonds among different materials can minimize energy losses, improve charge carrier transport, and consequently enhance the FF within the device. To quantify the interlayer anchoring strengths, researchers mainly rely on quantitative theoretical measurements, such as analyzing the binding energies of the layers involved. These measurements provide valuable insights into the stability and cohesion between the different layers, contributing to the design and development of solar cells with improved FF and enhanced overall efficiency. Similar to the VOC, FF is heavily related to interlayer interaction. Consequently, the location of passivation can be either at the adsorber-ETL or adsorber-HTL interface, as illustrated in Figure 4A.
Figure 4. (A) Illustration of the role of passivation layers on FF. The green arrow indicates the enhanced charge transfer rate due to the stronger interactions between different layers. (B) PDOS of the interface between (left) pristine, (middle) Sb3+ doped and (right) In3+doped Cs0.1FA0.9PbI3 perovskite films with TiO2[99]. Copyright 2018 Wiley. (C) Theoretical simulation of charge density. (left) The charge density at the interface between the Pb2+-based cluster, (middle) the In3+-based cluster, and (right) the Sb3+-based cluster (C) and the anatase TiO2 (101) surface, respectively[99]. Copyright 2018 Wiley. (D) Schematic diagram of band shift of perovskite and TiO2 in graded heterojunction structure with a mixed and graded interlayer. The negative and the positive circles represent electrons and holes, respectively, and the arrows express their flowing directions[99]. Copyright 2018 Wiley.
Passivation at the adsorber-ETL interface
Li et al. introduced phenethylammonium (PEA) cations, 4-Fluorophenethylammonium (F-PEA)), and 4-(Trifluoromethyl) phenethylammonium (CF3-PEA) with the distinct dipole moments to the interface between inorganic CsPbI3-xBrx perovskite light absorber layer and C60/ALD-SnO2 ETL[97]. Initially, they used the hybrid B3LYP DFT method with D3 vdW correction to calculate the electrostatic potentials of these passivators. Subsequently, DFT computations at the hybrid level with Heyd–Scuseria–Ernzerhof (HSE) functional and D3 vdW corrections were conducted. They found that CF3-PEA can have a stronger interaction with the light absorbers and C60 due to its high polarity. As a result, it significantly facilitated charge transfer between light absorbers and ETL, contributing to improving the FF from 80.7% to 82.8%.
Qiao et al. conducted a study to investigate the impact of Sb or In cation doping on Cs0.1FA0.9PbI3 PSCs, achieving an impressive FF of 84.0% by optimizing fabrication parameters[99]. To understand the underlying mechanisms, they performed theoretical analysis using DFT calculations at the PBE-GGA level with D3 vdW correction. Their theoretical analysis revealed that introducing Sb or In cations in the interlayers of the solar cell strengthened the interlayer interactions. The adsorption energies of PbI4, InI3, and SbI4 on the TiO2 ETL surface were determined to be -0.81, -0.89 and -0.90 eV, respectively, indicating the stability and favorable binding of the dopant cations to the TiO2 surface. This observation was supported by the corresponding I-Ti bond distances of 3.30, 3.28 and 3.25 Å when PbI4, InI3, and SbI4 were adsorbed, respectively. Further analysis of the partial DOS (PDOS) indicated a minimal overlap between Pb 6s and O 2p states, suggesting limited hybridization between these states. Similarly, weak hybridization was found between I 6p and Ti 3d states. However, in the interface with Sb or In cations, the analysis revealed enhanced hybridization between the cations' 5s orbitals and the O 2p orbitals within the antibonding energy range. Furthermore, the hybridization between the I 6p orbitals and the Ti 3d orbitals was strengthened after doping [Figure 4B]. This enhanced hybridization indicates a stronger interaction between the antimony or indium cations and the surrounding atoms. Charge density difference plots [Figure 4C] provided further evidence of the effects of doping, demonstrating increased charge transfer across the interface between the Sb cation-based cluster and the anatase TiO2 (101) surface. This stronger hybridization between the antimony or indium cations-based clusters and the TiO2 ETL surface greatly facilitates interface charge transfer, resulting in improved FF [Figure 4D]. The highest FF was observed in the Sb-doped PSC, reaching 84%. This was followed by an FF of 76% in the In-doped PSC. In comparison, the undoped Pb-based PSC exhibited an FF of 71%. The theoretical analysis shed light on the beneficial effects of Sb or In cation doping. These dopants improved interlayer interactions, resulting in improved charge transport and, ultimately, higher FF values in the fabricated PSCs.
Recently, Zhang et al. provided a guanidinium hydrochloride passivator that improves the FF[100]. The DFT results suggest that this passivator decreased the deep-level trap defects through the strong adsorption on the SnO2 ETL, such as the oxygen vacancy and unsaturated coordinated surface Sn atoms. Especially, the binding energy of the guanidinium hydrochloride on the SnO2 surface is 4.31 eV. Their DOS analysis demonstrates that both Sn 5p and Pb 6p states in guanidinium hydrochloride-passivated SnO2 and perovskite surfaces are similar to those in defect-free SnO2 and perovskite. Consequently, the passivation can reduce the trap density with reduced nonradiative recombination at this interface. This led to better electron transport with an improved FF of 81.81% from 77.72%.
Passivation at the adsorber-HTL interface
Liu et al. recently identified 1,3-bis(diphenylphosphino)propane (DPPP) as an effective passivator for improving the FF[101]. Employing DFT computations at the PBE-GGA level with the D3 vdW correction, the authors screened different Lewis bases and determined that P exhibited the highest binding affinity with the perovskite surface. Consequently, DPPP was selected as the promising passivators, demonstrating a tight interaction with surface Pb2+ with a binding energy of 2.24 eV. More importantly, DPPP exhibited a robust interaction with NiOx HTL, with the binding energy increasing to 4.31 eV upon spontaneous interaction with the perovskite surface and NiOx HTL. These strong interactions between the light absorber layer and HTL lead to an increased FF, observed in the experimentally from 79% to 82%. Fei et al. investigated the adsorption properties of bathocuproine (BCP) on the perovskite surface compared to the widely used dimethyl sulfoxide (DMSO) perovskite precursor solvent[102]. Utilizing the DFT computation at the PBE-GGA level with D3 vdW correction, they determined that BCP exhibited stronger adsorption, effectively disrupting the surface Pb-Pb dimer and eliminating associated deep gap states. Based on the DFT results, BCP was used as lead chelation molecules (LCMs) at the interface between the perovskite layer and PTAA HTLs. Competing with DMSO for lead ions through strong chelation, BCP reduced DMSO residue and the amorphous region in perovskites near the HTLs. Experimental validation confirmed the DFT predictions, revealing that replacing DMSO with BCP can reduce the amorphous region in perovskites near the HTLs, facilitating improved charge transfer. Consequently, the FF value increased from 80.3% to 82.5%.
When analyzing 2D materials, the adsorption is typically expressed in terms of single-layer adsorption rather than molecular adsorption. This means that the adsorption energy (Eads) is converted to the binding energy (ΔEb) by dividing it by the surface area (see Eq. 5). In the study conducted by Bati et al., they experimentally observed that the introduction of Cs+ dopants can increase the FF of the PSC with MXene from 71% to 76%[90]. Using the PBE-GGA method with the optB86b vdW correction, the DFT results provide theoretical insights into the role of Cs+ doping. It was found that Cs+ enhances the interaction strength between MXene and the perovskite film. The corresponding binding energy, indicating the strength of this interaction, is measured to be -0.016 eV/Å2, which is 23% higher than the system without Cs+. This increased interaction strength has led to the formation of a highly crystallized and improved morphology of the perovskite film. As a result, the defect density is effectively reduced. The stronger interaction between MXene and the perovskite film promotes charge transfer, thereby enhancing the FF values of the PSC.
PASSIVATION FOR IMPROVING STABILITY
The environmental stability of PSCs is a crucial aspect to consider, encompassing various factors. One area of foci for passivation techniques in perovskites is to improve their stability against moisture exposure. Physical surface passivation methods can be employed to isolate external layers, augmenting the hydrophobic properties of the cell to prevent water penetration and mitigate degradation. Additionally, cation doping serves as a strategy to enhance moisture resistance and overall stability in PSCs. Recent literature has highlighted several effective mechanisms to improve surface protection and enhance environmental stability. These mechanisms provide insights into strategies for preserving the integrity and performance of PSCs in challenging environmental conditions. By addressing issues related to moisture exposure, researchers aim to enhance the LTS and durability of PSCs for practical applications.
Yang et al. used the optB86b method with vdW correction to investigate the blocking effect of specific bulky organic cations on water pathways, revealing a prevention mechanism for water adsorption on the
Figure 5. (A) Water molecule adsorption on perovskite surfaces. The MA (top) and TEA surfaces (bottom) are terminated with methyl and tetra-ethyl ammonium cations, respectively[105]. Copyright 2016 Nature Publishing Group. (B) Modeling atomic structures of functionalized perovskite surfaces. Side (top) and top (middle) views, respectively, of the optimized geometries of the (100) surfaces of MA, TMA and TEA samples. Calculated atomic structure (side view) of different (100) surfaces with molecularly adsorbed water (bottom)[105]. Copyright 2016 Nature Publishing Group. (C) Water absorption ratio of the perovskite crystals under a relative humidity of 90% ± 5% and dark conditions. Samples of more than 5 g were used in all tests for accuracy. Three regions are defined to classify the moisture resistance of all the samples[105]. Copyright 2016 Nature Publishing Group. (D) Atomic structures of Cs1-xFAxPbI3 (001) surfaces with adsorbed H2O and C4H6O2 molecules in a unit cell: CsPbI3 (left); Cs0.5FA0.5PbI3 (middle); and FAPbI3 (right)[64]. Copyright 2020 Royal Society of Chemistry. (E) Evolution of normalized absorbance for pristine (green) and chelated (purple, Pb(DDTC)2) CsPbI2Br thin films under dark in ambient atmosphere (RH, 15% ± 3%)[106]. Copyright 2020 Nature Publishing Group.
Zhang et al. employed DFT calculations at the PBE-GGA level, incorporating the Tkatchenko-Scheffler vdW correction method, to explore the adsorption behavior of the phosphorene monolayer on the MAPbI3 (001) surface[107]. They specifically examined two surface terminations: PbI-termination and bare-cation termination. Their findings indicated a stronger interaction between phosphorene and the PbI-terminated surface compared to the bare cation termination. This enhanced interaction was attributed to the larger attractions between the electron-rich phosphorus (P) atom in phosphorene and the electrophilic Pb atom in the perovskite surface. To evaluate the stability of perovskite materials protected by a phosphorene passivation layer, classical molecular dynamics (MD) simulations were employed. The MD results demonstrated that water molecules could easily approach both the PbI-terminated and cation-terminated perovskite surfaces. However, the surface passivation provided by phosphorene effectively blocked the adsorption of water molecules. This blocking effect can be attributed to the hydrophobic nature of phosphorene, which is consistent with experimental observations. This study highlighted the potential of phosphorene as a passivation layer to enhance the stability of perovskite materials by preventing water adsorption.
CONCLUSION AND REMAINING CHALLENGES
Passivation plays a crucial role in advancing efficient PSCs, aiming to enhance various aspects of their performance. Effective passivation mechanisms contribute to improved exciton transport, recombination prevention, and interlayer interactions. Progress has been notable in dopant, interfacial, and surface passivation techniques. Ongoing research focuses on refining passivation mechanisms, including exciton transport improvement, recombination prevention, interlayer anchoring, and moisture protection. A comprehensive understanding at the atomic scale is crucial for optimizing passivation strategies. This includes investigating the interactions between the passivator and perovskite material to improve VOC, JSC, and FF while exploring stability mechanisms to maintain high performance over time. Based on the recent theoretical studies, the selection criteria for a good passivator for PSCs from DFT computations can be summarized as follows:
i. Improvement of VOC: The ideal passivator should align its CBM and VBM locations with the band structures of the perovskite light absorbers, ETL, and HTL. Additionally, the passivator should effectively suppress defect states, optimizing the band alignment between the perovskite light absorbers and charge transfer layers.
ii. Improvement of JSC: Passivators should possess small charge-effective masses to facilitate efficient charge transfer. They should also exhibit low absorption and reflectivity coefficients in the visible light range, enabling a higher amount of incident light to be absorbed and utilized by the light absorbers.
iii. Improvement of FF: The passivator can enhance interlayer interactions between different layers with the PSC devices, minimizing energy losses and improving charge carrier transport.
iv. Enhancement of stability: LTS and resistance to degradation are crucial, ensuring the passivator maintains its effectiveness throughout the lifetime of PSCs. Passivators should have stronger binding strength with the light absorbers to provide a protective barrier to safeguard the perovskite material.
The passivation process is important as it can improve the critical parameters associated with the best PSC efficiency. The combined effectiveness of these properties determines a successful passivator. The examples in this review are summarized in Table 1, showing that passivators can improve VOC, JSC, and FF to some extent. Some passivators can have a specific impact on a specific parameter, and all of them can significantly enhance the LTS. DFT studies can provide a theoretical explanation of these improvements. To understand the different influences of passivators, corresponding XC functionals were used with the compromise between the computational cost and accuracy.
Summary of the function of the different passivators for specific perovskite materials on the open-circuit voltage (VOC), short-circuit current density (JSC), fill factor (FF) and long-term stability (LST) with the corresponding exchange-correlation (XC) functionals
Perovskite | Passivator(s) | XC | VOC | JSC | FF | LTS | Ref. |
CsPbI3 | Benzoyl hydrazine | PBE, D3 | 2.6% | 1.9% | 1.6% | √ | [84] |
Rb0.05Cs0.05(FA0.83MA0.17)Pb(I0.83Br0.17)3 | acetylcholine | PBE | 8.0% | 3.8% | √ | [85] | |
(FAPbI3)0.95(MAPbBr3)0.05 | chlorobenzenesulfonic potassium salts | PBE, D3 | 5.7% | √ | [86] | ||
MAPbI3 | Single-walled carbon nanotubes | PBE | 8.0% | 23% | √ | [87] | |
Rb0.05Cs0.05MA0.05FA0.85Pb(I0.95Br0.05)3 | 3-(amino methyl) pyridine | GGA | 3.8% | 1.6% | 3.6% | √ | [89] |
MAPbI3 | Cs-doped Ti3C2Tx | optB86b | 2.7% | 3.9% | 7.0% | √ | [90] |
CsPbI3-xBrx | Boc-S-4-methoxy-benzyl-L-cysteine | PBE | 9.2% | 2.8% | 4.4% | √ | [91] |
CsPbI3-xBrx | trifluoroacetamidine | PBE | 3.5% | 2.5% | 5.3% | √ | [92] |
CsPbI3-xBrx | 4-Fluorophenethyl ammonium, 4-(Trifluoromethyl) phenethylammonium | B3LYP, HES, D3 | 19% | 2.3% | 4.4% | √ | [97] |
CsPbI3-xBrx | 2-mercapto-1-methylimidazole | PBE | 11% | 2.8% | √ | [98] | |
Cs0.1FA0.9 PbI3 | Sb dopants In dopants | PBE, D3 | 7.8% | 14% | 17% | [99] | |
FAPbI3 | bathocuproine | PBE, D3 | 1.2% | 3.5% | 3.1% | √ | [102] |
MAPbI3 | tris(2,4,6-trichloro phenyl) methyl | PBE, D3 | 3.7% | 5.1% | 4.5% | √ | [103] |
(FAPbI3)0.95(MAPbBr3)0.05 | OATsO | PBEsol, D3 | 4.9% | 4.9% | √ | [104] | |
MAPbI3 | TEA | optB86b, vdW-DF | √ | [105] | |||
CsPbI2Br | dithiocarbamate | GGA, D3, SOC | 13% | 4.2% | √ | [106] |
While the computational results can reproduce a qualitative trend along with the experimental observations, which were provided throughout this review, the theoretical results cannot provide a quantitative comparison with the experimental data due to the oversimplified models used in theoretical studies without the consideration of most of the working conditions. Additionally, while DFT-based calculation methods offer a means to uncover atomic-level details of passivation within the solar cell systems, the extension of the molecular-scale models to macroscopic descriptions related to solar-to-electricity processes is required. For example, the grain effect in systems with large numbers of atoms and small grains can result in increased surface area and surface states that require consideration. Consequently, a multi-scale simulation approach is required, encompassing atomic-level quantum states and the macroscopic observations of passivation technologies. There are two main categories of multi-scale simulation approaches: concurrent and sequential. Concurrent multi-scale modeling involves directly applying multiple levels of theory in a single simulation, explicitly capturing phenomena occurring across various time and length scales. A common example of concurrent coupling in electrochemistry is quantum mechanics/molecular mechanics (QM/MM) modeling, where the electronic structure method describes a small portion of reactions while molecular force fields are employed for the dynamical part. In contrast, sequential multi-scale modeling involves using results from one level of modeling as input for another level, allowing subsequent simulations to overcome the time and length scale limitations of the parent model. This approach, known as parameter passing, enables more flexibility in the simulations. It is important to note that while these approaches offer computational efficiency, there are trade-offs to consider.
DECLARATIONS
Authors’ contributions
Conceptualization, investigation, methodology, validation, formal analysis, writing, reviewing, and editing: Allen OJ
Methodology, validation, formal analysis, writing, reviewing, and editing: Kang J
Figure generation, validation, formal analysis: Qian S
Writing, reviewing, and editing: Zhang L, Hinsch JJ
Conceptualization, validation, supervision, writing, reviewing, and editing: Wang Y
Availability of data and materials
Not applicable.
Financial support and sponsorship
The authors acknowledge financial support from Griffith University (CEE2550), the Australian Research Council Discovery Project (Grant No. DP210103266), and the Discovery Early Career Researcher Award (DE240101090).
Conflicts of interest
All authors declared that there are no conflicts of interest.
Ethical approval and consent to participate
Not applicable.
Consent for publication
Not applicable.
Copyright
© The Author(s) 2024.
REFERENCES
1. Jesper Jacobsson T, Correa-baena J, Pazoki M, et al. Exploration of the compositional space for mixed lead halogen perovskites for high efficiency solar cells. Energy Environ Sci 2016;9:1706-24.
3. Tan Q, Li Z, Luo G, et al. Inverted perovskite solar cells using dimethylacridine-based dopants. Nature 2023;620:545-51.
4. Yang Z, Zhang W, Wu S, et al. Slot-die coating large-area formamidinium-cesium perovskite film for efficient and stable parallel solar module. Sci Adv 2021;7:eabg3749.
5. Yang F, Jang D, Dong L, et al. Upscaling solution-processed perovskite photovoltaics. Adv Energy Mater 2021;11:2101973.
6. Gao F, Zhao Y, Zhang X, You J. Recent progresses on defect passivation toward efficient perovskite solar cells. Adv Energy Mater 2020;10:1902650.
7. Ball JM, Petrozza A. Defects in perovskite-halides and their effects in solar cells. Nat Energy 2016;1:16149.
8. You S, Eickemeyer FT, Gao J, et al. Bifunctional hole-shuttle molecule for improved interfacial energy level alignment and defect passivation in perovskite solar cells. Nat Energy 2023;8:515-25.
9. Meng L, You J, Yang Y. Addressing the stability issue of perovskite solar cells for commercial applications. Nat Commun 2018;9:5265.
10. Wang R, Mujahid M, Duan Y, Wang Z, Xue J, Yang Y. A review of perovskites solar cell stability. Adv Funct Mater 2019;29:1808843.
11. Li Z, Li B, Wu X, et al. Organometallic-functionalized interfaces for highly efficient inverted perovskite solar cells. Science 2022;376:416-20.
12. Abdi-Jalebi M, Andaji-Garmaroudi Z, Cacovich S, et al. Maximizing and stabilizing luminescence from halide perovskites with potassium passivation. Nature 2018;555:497-501.
13. Zhan Y, Yang F, Chen W, et al. Elastic lattice and excess charge carrier manipulation in 1D-3D Perovskite solar cells for exceptionally long-term operational stability. Adv Mater 2021;33:e2105170.
14. Luo L, Zeng H, Wang Z, et al. Stabilization of 3D/2D perovskite heterostructures via inhibition of ion diffusion by cross-linked polymers for solar cells with improved performance. Nat Energy 2023;8:294-303.
15. Li Y, Liu L, Zheng C, et al. Plant-derived l -theanine for ultraviolet/ozone resistant perovskite photovoltaics. Adv Energy Mater 2023;13:2203190.
16. Park J, Kim J, Yun HS, et al. Controlled growth of perovskite layers with volatile alkylammonium chlorides. Nature 2023;616:724-30.
17. Li M, Sun R, Chang J, et al. Orientated crystallization of FA-based perovskite via hydrogen-bonded polymer network for efficient and stable solar cells. Nat Commun 2023;14:573.
18. Zhang Z, Qiao L, Meng K, Long R, Chen G, Gao P. Rationalization of passivation strategies toward high-performance perovskite solar cells. Chem Soc Rev 2023;52:163-95.
19. Xia J, Liang C, Gu H, et al. Surface passivation toward efficient and stable perovskite solar cells. Energy Environ Mater 2023;6:e12296.
20. Azaid A, Kacimi R, Alaqarbeh M, et al. Design of a D-Di-π-A architecture with different auxiliary donors for dye-sensitized solar cells: density functional theory/time-dependent-density functional theory study of the effect of secondary donors. Advcd Theory Sims 2023;6:2300054.
21. Hassan T, Adnan M, Hussain R, Hussain F, Khan MU. Molecular engineering of Pyran-fused acceptor-donor-acceptor-type non-fullerene acceptors for highly efficient organic solar cells - a density functional theory approach. J Phys Org Chem 2023;36:e4507.
22. Kagdada HL, Roondhe B, Roondhe V, et al. Exploring a-site cation variations in dion-jacobson two-dimensional halide perovskites for enhanced solar cell applications: a density functional theory study. Adv Energy Sustain Res 2024;5:2300147.
23. Saloni S, Ranjan P, Chakraborty T. A computational study of CuCrX2 (X = S, Se, Te) for intermediate band solar cell: conceptual density functional theory approach. J Mol Graph Model 2023;124:108534.
24. Setsoafia DDY, Ram KS, Mehdizadeh-rad H, Ompong D, Singh J. Density functional theory simulation of optical and photovoltaic properties of DRTB-T donor-based organic solar cells. Int J Energy Res 2023;2023:1-12.
25. Srivastava A, Lenka TR, Anthoniappen J, Tripathy SK. Investigation on thermodynamic properties of novel Ag2SrSn(S/Se)4 quaternary chalcogenide for solar cell applications: a density functional theory study. In: Lenka TR, Misra D, Fu L, editors. Micro and nanoelectronics devices, circuits and systems. Singapore: Springer Nature; 2023. pp. 103-10.
26. Taouali W, Alimi K, Sindhoo Nangraj A, Casida ME. Density-functional theory (DFT) and time-dependent DFT study of the chemical and physical origins of key photoproperties of end-group derivatives of a nonfullerene acceptor molecule for bulk heterojunction organic solar cells. J Comput Chem 2023;44:2130-48.
27. Kaiser W, Carignano M, Alothman AA, et al. First-principles molecular dynamics in metal-halide perovskites: contrasting generalized gradient approximation and hybrid functionals. J Phys Chem Lett 2021;12:11886-93.
28. Ohto T, Dodia M, Imoto S, Nagata Y. Structure and dynamics of water at the water-air interface using first-principles molecular dynamics simulations within generalized gradient approximation. J Chem Theory Comput 2019;15:595-602.
29. Pandech N, Kongnok T, Palakawong N, Limpijumnong S, Lambrecht WRL, Jungthawan S. Effects of the van der Waals interactions on structural and electronic properties of CH3NH3(Pb,Sn)(I,Br,Cl)3 halide perovskites. ACS Omega 2020;5:25723-32.
30. Wang Y, de Gironcoli S, Hush NS, Reimers JR. Successful a priori modeling of CO adsorption on Pt(111) using periodic hybrid density functional theory. J Am Chem Soc 2007;129:10402-7.
31. Gusakova J, Wang X, Shiau LL, et al. Electronic properties of bulk and monolayer TMDs: theoretical study within DFT framework (GVJ-2e Method). Physica Status Solidi 2017;214:1700218.
32. Borlido P, Aull T, Huran AW, Tran F, Marques MAL, Botti S. Large-scale benchmark of exchange-correlation functionals for the determination of electronic band gaps of solids. J Chem Theory Comput 2019;15:5069-79.
33. Wang Y, Zhang H, Liu P, Yao X, Zhao H. Engineering the band gap of bare titanium dioxide materials for visible-light activity: a theoretical prediction. RSC Adv 2013;3:8777-82.
34. Zaki NH, Ali AMM, Mohamad Taib MF, Wan Ismail WIN, Sepeai S, Ramli A. Dispersion-correction density functional theory (DFT+D) and spin-orbit coupling (SOC) method into the structural, electronic, optical and mechanical properties of CH3NH3PbI3. Comput Condens Matter 2023;34:e00777.
35. Shi T, Yin W, Hong F, Zhu K, Yan Y. Unipolar self-doping behavior in perovskite CH3NH3PbBr3. Appl Phys Lett 2015;106:103902.
36. Huang Y, Sun QD, Xu W, He Y, Yin WJ. Halide perovskite materials for solar cells: a theoretical review. Acta Phys Sin 2017;33:1730-51.
37. Yin W, Yang J, Kang J, Yan Y, Wei S. Halide perovskite materials for solar cells: a theoretical review. J Mater Chem A 2015;3:8926-42.
38. Amat A, Mosconi E, Ronca E, et al. Cation-induced band-gap tuning in organohalide perovskites: interplay of spin-orbit coupling and octahedra tilting. Nano Lett 2014;14:3608-16.
39. Bhattacharya S, Kanai Y. Spin-orbit-coupling-induced band splitting in two-dimensional hybrid organic-inorganic perovskites: Importance of organic cations. Phys Rev Mater 2023;7:055001.
40. Ronca E, De Angelis F, Fantacci S. Time-dependent density functional theory modeling of spin-orbit coupling in ruthenium and osmium solar cell sensitizers. J Phys Chem C 2014;118:17067-78.
41. Even J, Pedesseau L, Jancu J, Katan C. Importance of spin-orbit coupling in hybrid organic/inorganic perovskites for photovoltaic applications. J Phys Chem Lett 2013;4:2999-3005.
42. Idrissi S, Labrim H, Bahmad L, Benyoussef A. DFT and TDDFT studies of the new inorganic perovskite CsPbI3 for solar cell applications. Chem Phys Lett 2021;766:138347.
43. Das T, Di Liberto G, Pacchioni G. Density functional theory estimate of halide perovskite band gap: when spin orbit coupling helps. J Phys Chem C 2022;126:2184-98.
44. Alsalamah IM, Shaari A, Alsaif NA, Yamusa SA, Lakshminarayana G, Rekik N. Exploring the structural properties and the optoelectronic features of RbPbX3 (X = Cl, F) perovskite crystals for solar cells solicitations: showcasing the DFT predictions. Chem Phys 2023;573:111978.
45. Borges-martínez M, Saavedra-torres M, Schott E, Zarate X. Computational design and properties elucidation of new (FAPbI3)1-x-y(MAPbBr3)y(CsPbBr3)x photoactive systems for their application in perovskite solar cells. Mater Today Commun 2023;34:105324.
46. Arfaoui Y, Khenfouch M, Habiballah N. A DFT and time-dependent DFT investigation of the structural, electronic and optical properties of lead-free FAMgI3 perovskite for photovoltaic applications. J Electron Mater 2024;53:881-90.
47. Glockzin B, Oakley MS, Karmakar A, et al. Alkali tin halides: exploring the local structure of A2SnX6 (A = K, Rb; X = Cl, Br, I) compounds using solid-state NMR and DFT computations. J Phys Chem C 2023;127:7284-98.
48. Graupner DR, Kilin DS. Size effects on polaron formation in lead chloride perovskite thin films. Mol Phys 2023.
49. Haroon M, Baig MW, Akhtar T, Tahir MN, Ashfaq M. Relativistic two-component time dependent density functional studies and Hirshfeld surface analysis of halogenated arylidenehydrazinylthiazole derivatives. J Mol Structure 2023;1287:135692.
50. Idrissi S, Mounkachi O, Bahmad L, Benyoussef A. Study of the solar perovskites: XZnF3 (X = Ag, Li or Na) by DFT and TDDFT methods. J Korean Ceram Soc 2023;60:424-33.
51. Islam MR, Mazumder AAM, Mojumder MRH, Shifat ASMZ, Hossain MK. Strain-induced tunable optoelectronic properties of inorganic halide perovskites APbCl3 (A = K, Rb, and Cs). Jpn J Appl Phys 2023;62:011002.
52. Javed M, Sattar MA, Benkraouda M, Amrane N, Najar A. Strained induced metallic to semiconductor transitions in 2D Ruddlesden Popper perovskites: a GGA + SOC approach. Appl Surf Sci 2023;627:157244.
53. Kumar D, Chand P. Enhanced optical and thermoelectric properties of Ti doped half - Heusler alloy NbRuP: a first principles study. Solid State Commun 2023;366-7:115179.
54. Kumar G, Ravidas BK, Bhattarai S, Roy MK, Samajdar DP. Exploration of the photovoltaic properties of oxide-based double perovskite Bi2FeCrO6 using an amalgamation of DFT with spin-orbit coupling effect and SCAPS-1D simulation approaches. New J Chem 2023;47:18640-58.
55. Laghzaoui S, Lamrani AF, Laamara RA. Robust half-metallic ferromagnet in doped double perovskite Sr2TiCoO6 by rare-earth elements for photovoltaic and thermoelectric conversion: a DFT method. J Phys Chem Solid 2023;183:111639.
56. Li S, Chen Y, Wang Z, et al. Theoretical studies of new iridium-based terpolymer donors for high-efficiency triplet-material-based organic photovoltaics: Incorporation of different iridium(III) complexes. Mater Chem Phys 2023;302:127780.
57. Moaddeli M, Kanani M, Grünebohm A. Electronic and structural properties of mixed-cation hybrid perovskites studied using an efficient spin-orbit included DFT-1/2 approach. Phys Chem Chem Phys 2023;25:25511-25.
58. Mokkath J. Tailoring the infrared resonances of sulfide perovskites. Mater Today Chem 2023;30:101589.
59. Muthumari M, Manjula M, Veluswamy P, Kuznetsov DV. First principles calculations to investigate structural, electronic, mechanical, thermoelectric and optical properties of Bi- and Se-doped SnTe. J Phys Chem Solid 2023;176:111232.
60. Rahman MF, Rahman MA, Islam MR, et al. Unraveling the strain-induced and spin-orbit coupling effect of novel inorganic halide perovskites of Ca3AsI3 using DFT. AIP Adv 2023;13:085329.
61. Raju N, Tripathi D, Lahiri S, Thangavel R. Heat reflux sonochemical synthesis of Cu3BiS3 quantum dots: experimental and first-principles investigation of spin-orbit coupling on structural, electronic, and optical properties. Solar Energy 2023;259:107-18.
62. Supatutkul C, Sitarachu K, Laosiritaworn Y, Jaroenjittichai AP. Quasiparticle band structures of Cs2B+B3+Br6 lead-free halide double perovskites. Mater Today Commun 2023;36:106751.
63. Yami NFNA, Ramli A, Nawawi WI, et al. Structural, electronic, and optical properties of lower-dimensional hybrid perovskite lead-iodide frameworks + SOC via density functional theory. Emergent Mater 2023;6:999-1007.
64. Liu J, Kang J, Chen S, et al. Effects of compositional engineering and surface passivation on the properties of halide perovskites: a theoretical understanding. Phys Chem Chem Phys 2020;22:19718-24.
65. Heyd J, Scuseria GE, Ernzerhof M. Erratum: “Hybrid functionals based on a screened Coulomb potential”. J Chem Phys 2006;124:219906.
66. West AR. Solid state chemistry and its applications. 2nd ed. Hoboken: John Wiley & Sons; 2022.
67. Zhilyakov LA, Kostanovskii AV, Pokhil GP. Condition of formation of 2D coulomb crystal on the surface of dielectric. High Temp 2008;46:721-4.
68. Umari P, Mosconi E, De Angelis F. Relativistic GW calculations on CH3NH3PbI3 and CH3NH3SnI3 perovskites for solar cell applications. Sci Rep 2014;4:4467.
69. Li H, Shi J, Deng J, et al. Intermolecular π-π conjugation self-assembly to stabilize surface passivation of highly efficient perovskite solar cells. Adv Mater 2020;32:e1907396.
70. Zheng Y, Zhang S, Ma J, et al. Codependent failure mechanisms between cathode and anode in solid state lithium metal batteries: mediated by uneven ion flux. Sci Bull 2024;69:317-9.
71. Zhao H, Kordas K, Ojala S. Recent advances in synthesis of water-stable metal halide perovskites and photocatalytic applications. J Mater Chem A 2023;11:22656-87.
72. Goyal A, Singh PP, Mondal T. Investigating the role of Co and Fe in bimetallic perovskite catalysts (LaNiO3) for steam reforming of Bio-Oil model oxygenates: a DFT study. Carbon 2023;2:2.606. Available from: https://oxford-abstracts.s3.amazonaws.com/f0e3a240-bf65-43be-8a7c-c42d93fa4e3e.pdf [Last accessed on 22 Apr 2024].
73. Bayendang NP, Kahn MT, Balyan V. Thermoelectric generators (TEGs) modules-optimum electrical configurations and performance determination. AIMS Energy 2022;10:102-30.
74. Imai Y, Nishizawa S, Ito K. Reduction of LSI maximum power consumption with standard cell library of stack structured cells. IEICE Trans Fund 2022;E105.A:487-96.
75. Keller J, Aboulfadl H, Stolt L, Donzel-gargand O, Edoff M. Rubidium fluoride absorber treatment for wide-gap (Ag,Cu)(In,Ga)Se2 solar cells. Solar RRL 2022;6:2200044.
76. Kulkarni V, Ghaisas G, Krishnan S. Performance analysis of an integrated battery electric vehicle thermal management. J Energy Stor 2022;55:105334.
77. Okedu KE, Al Ghaithi ASS. Comparative study of the internal dynamic failures of grid-connected solar PVs: the case of the oman power network. Front Energy Res 2022;10:858803.
78. Mozaffari S, Kiamehr Z. A theoretical study on internal losses of heat generation in inorganic metal oxide charge transporting layers-based inverted PSC. Opt Quant Electron 2023;55:826.
79. Li H, Shi P, Wang L, et al. Cooperative catalysis of polysulfides in lithium-sulfur batteries through adsorption competition by tuning cationic geometric configuration of dual-active sites in spinel oxides. Angew Chem Int Ed 2023;62:e202216286.
80. Xia J, Sohail M, Nazeeruddin MK. Efficient and stable perovskite solar cells by tailoring of interfaces. Adv Mater 2023;35:e2211324.
81. Pratheek M, Abhinav T, Bhattacharya S, Chandra GK, Predeep P. Recent progress on defect passivation in perovskites for solar cell application. Mater Sci Energy Technol 2021;4:282-9.
82. Zhu R, Guan N, Wang D, Bao Y, Wu Z, Song L. Review of defect passivation for NiOx-based inverted perovskite solar cells. ACS Appl Energy Mater 2023;6:2098-121.
83. Zhang Y, Liu Y, Liu S. Composition engineering of perovskite single crystals for high-performance optoelectronics. Adv Funct Mater 2023;33:2210335.
84. Che Y, Liu Z, Duan Y, et al. Hydrazide derivatives for defect passivation in pure CsPbI3 Perovskite Solar Cells. Angew Chem Int Ed 2022;61:e202205012.
85. Zhang Z, Jiang J, Xiao Liu X, et al. Surface-anchored acetylcholine regulates band-edge states and suppresses ion migration in a 21%-efficient quadruple-cation perovskite solar cell. Small 2022;18:e2105184.
86. Dong Y, Shen W, Dong W, et al. Chlorobenzenesulfonic potassium salts as the efficient multifunctional passivator for the buried interface in regular perovskite solar cells. Adv Energy Mater 2022;12:2200417.
87. Batmunkh M, Macdonald TJ, Shearer CJ, et al. Carbon nanotubes in TiO2 nanofiber photoelectrodes for high-performance perovskite solar Cells. Adv Sci 2017;4:1600504.
88. Chavan RD, Parikh N, Tavakoli MM, et al. Band alignment and carrier recombination roles on the open circuit voltage of ETL-passivated perovskite photovoltaics. Intl J Energy Res 2022;46:6022-30.
89. Jiang Q, Tong J, Xian Y, et al. Surface reaction for efficient and stable inverted perovskite solar cells. Nature 2022;611:278-83.
90. Bati AS, Sutanto AA, Hao M, et al. Cesium-doped Ti3C2Tx MXene for efficient and thermally stable perovskite solar cells. Cell Rep Phys Sci 2021;2:100598.
91. Zhang H, Tian Q, Xiang W, et al. Tailored cysteine-derived molecular structures toward efficient and stable inorganic perovskite solar cells. Adv Mater 2023;35:e2301140.
92. Zhang H, Xiang W, Zuo X, et al. Fluorine-containing passivation layer via surface chelation for inorganic perovskite solar cells. Angew Chem Int Ed 2023;62:e202216634.
93. Batmunkh M, Vimalanathan K, Wu C, et al. Efficient production of phosphorene nanosheets via shear stress mediated exfoliation for low-temperature perovskite solar cells. Small Method 2019;3:1800521.
94. Macdonald TJ, Clancy AJ, Xu W, et al. Phosphorene nanoribbon-augmented optoelectronics for enhanced hole extraction. J Am Chem Soc 2021;143:21549-59.
95. Allen OJ, Kang J, Wang Y. First-principles study of group VA monolayer passivators for perovskite solar cells. ACS Appl Nano Mater 2023;6:4279-87.
96. Cheng L, Zhang C, Liu Y. The Optimal electronic structure for high-mobility 2D semiconductors: exceptionally high hole mobility in 2D antimony. J Am Chem Soc 2019;141:16296-302.
97. Li T, Xu J, Lin R, et al. Inorganic wide-bandgap perovskite subcells with dipole bridge for all-perovskite tandems. Nat Energy 2023;8:610-20.
98. Xu T, Xiang W, Yang J, et al. Interface modification for efficient and stable inverted inorganic perovskite solar cells. Adv Mater 2023;35:e2303346.
99. Qiao HW, Yang S, Wang Y, et al. A gradient heterostructure based on tolerance factor in high-performance perovskite solar cells with 0.84 fill factor. Adv Mater 2019;31:e1804217.
100. Zhang B, Oh J, Sun Z, et al. Buried guanidinium passivator with favorable binding energy for perovskite solar cells. ACS Energy Lett 2023;8:1848-56.
101. Liu Q, Wu Y, Li D, et al. Dilute alloying to implant activation centers in nitride electrocatalysts for lithium-sulfur batteries. Adv Mater 2023;35:e2209233.
102. Fei C, Li N, Wang M, et al. Lead-chelating hole-transport layers for efficient and stable perovskite minimodules. Science 2023;380:823-9.
103. Xie P, Xiao H, Qiao Y, et al. Radical reinforced defect passivation strategy for efficient and stable MAPbI3 perovskite solar cells fabricated in air using a green anti-solvent process. Chem Eng J 2023;462:142328.
104. Tan S, Huang T, Yavuz I, et al. Stability-limiting heterointerfaces of perovskite photovoltaics. Nature 2022;605:268-73.
105. Yang S, Wang Y, Liu P, Cheng Y, Zhao HJ, Yang HG. Functionalization of perovskite thin films with moisture-tolerant molecules. Nat Energy 2016;1:15016.
106. He J, Liu J, Hou Y, Wang Y, Yang S, Yang HG. Surface chelation of cesium halide perovskite by dithiocarbamate for efficient and stable solar cells. Nat Commun 2020;11:4237.
Cite This Article
Export citation file: BibTeX | RIS
OAE Style
Allen OJ, Kang J, Qian S, Hinsch JJ, Zhang L, Wang Y. A theoretical review of passivation technologies in perovskite solar cells. Energy Mater 2024;4:400037. http://dx.doi.org/10.20517/energymater.2023.111
AMA Style
Allen OJ, Kang J, Qian S, Hinsch JJ, Zhang L, Wang Y. A theoretical review of passivation technologies in perovskite solar cells. Energy Materials. 2024; 4(3): 400037. http://dx.doi.org/10.20517/energymater.2023.111
Chicago/Turabian Style
Allen, Oscar J., Jian Kang, Shangshu Qian, Jack J. Hinsch, Lei Zhang, Yun Wang. 2024. "A theoretical review of passivation technologies in perovskite solar cells" Energy Materials. 4, no.3: 400037. http://dx.doi.org/10.20517/energymater.2023.111
ACS Style
Allen, OJ.; Kang J.; Qian S.; Hinsch JJ.; Zhang L.; Wang Y. A theoretical review of passivation technologies in perovskite solar cells. Energy Mater. 2024, 4, 400037. http://dx.doi.org/10.20517/energymater.2023.111
About This Article
Special Issue
Copyright
Data & Comments
Data
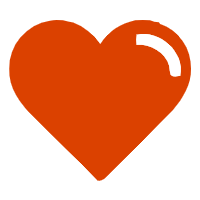

Comments
Comments must be written in English. Spam, offensive content, impersonation, and private information will not be permitted. If any comment is reported and identified as inappropriate content by OAE staff, the comment will be removed without notice. If you have any queries or need any help, please contact us at support@oaepublish.com.