Detecting flap compromise: an updated review of techniques to monitor microsurgical flaps post-operatively in breast reconstruction
Abstract
Breast reconstructive surgery utilizing free tissue transfer has revolutionized the restoration of aesthetic and functional outcomes for patients. Even for the most routine free flap procedures, substantial hospital resources and costs are necessary. The effectiveness of free flap surgery, along with any reconstructive procedure, hinges upon meticulous patient selection, thorough pre-operative planning, well-informed peri-operative decision-making, and diligent post-operative monitoring and care for the patient. This article presents a review of standard clinical care monitoring techniques during the post-operative period, as well as the diverse strategies currently employed for post-operative flap monitoring.
Keywords
INTRODUCTION
Microvascular free flap surgery represents an innovative reconstructive technique that has greatly broadened the possibilities of surgical breast reconstruction[1-3]. Recently, microsurgery has seen a surge in popularity, accompanied by the introduction of new flap types and expanded indications for their use[4]. Nonetheless, the occurrence of flap loss remains a dreaded outcome, with reported failure rates ranging from 2% to 5%[5-7]. The considerable effort, time, and cost invested in microvascular breast reconstruction, spanning from pre-operative planning to post-operative follow-up, intensify the impact of such losses. Pre-operative planning, including possible imaging, may begin several months in advance. Post-operatively, patients are admitted to the intensive care unit (ICU) for hourly flap checks during the first 48 hours, when the flap is most vulnerable[8]. However, evidence has emerged suggesting that patients can be safely managed in step-down units instead of ICUs, allowing for accelerated discharges without an increased risk of flap loss[9]. Nevertheless, the costs associated with autologous breast reconstruction remain high. According to the Healthcare Cost and Utilization Project National Inpatient Sample dataset, the average cost of a deep inferior epigastric artery perforator (DIEP) flap for autologous breast reconstruction amounts to $22,677[10]. Flap failure amplifies this already high cost by 50% to 77%, primarily due to extended hospital stays and the need for secondary operations, thereby further compromising aesthetic and/or functional outcomes for patients[11,12].
Free flaps fail when tissue perfusion is compromised and unable to meet the metabolic demands of the tissue. This occurs from inadequate inflow, inadequate outflow, or intrinsic issues. Among these causes, venous insufficiency is the most frequently encountered, as veins are delicate structures prone to compression or damage from trauma or pressure[13-15]. Certain intraoperative decisions can increase the post-operative risk to the vasculature, such as the presence of perforators with long or tortuous courses. Additionally, elevated tissue pressure, resulting from factors like edema, hematoma, or external compression (e.g., positioning), can surpass perfusion pressure. Certain patient factors (e.g., age, disease, body weight, smoking, pharmacological history), as well as the specific flap type, can further contribute to the risk of experiencing any of these causes[16].
The initial 48-hour period following surgery poses the highest risk for flap failure, emphasizing the importance of employing sensitive strategies for early detection. Timely identification of flap failure can be instrumental in salvaging compromised flaps by prompting an urgent return to the operating room for diagnostic assessment and salvage attempts[8,17-21]. Flaps can be successfully salvaged in 28% to 90% of cases, but this range is highly dependent on the time of detection and take-back[22-25]. As the field of breast reconstruction continues to expand with various options, the challenge of early detection has necessitated the concurrent evolution of advanced flap monitoring techniques. For example, the introduction of nipple-sparing mastectomy, which may involve immediate reconstruction using a buried free flap without a skin paddle, requires the development of reliable monitoring methods independent of cutaneous visualization. In addition to thorough clinical examinations conducted by experienced professionals, several adjunctive post-operative monitoring technologies have been developed to complement physical examinations and contribute to reducing failure rates. This narrative review summarizes the techniques employed to facilitate early detection of threatened flaps in breast reconstruction, encompassing post-operative protocols, clinical examinations, and supplemental technologies. We also highlight novel advancements and future directions in plastic and reconstructive surgery.
POST-OPERATIVE FLAP MONITORING
Most institutions follow specific protocols to ensure standardized care during the vulnerable post-operative period (48 h), typically in the ICU with trained staff and immediate access to the operating room if indicated. However, protocols vary significantly between institutions and flap types, and there remains no national consensus on the frequency or duration of monitoring. Recent discussions have emerged regarding the necessity of ICU monitoring for free flaps and the utility of monitoring beyond the initial 48 h[26,27]. A meta-analysis of head and neck microvascular reconstruction indicated that immediate post-operative ICU care did not lead to a decrease in flap failure or complication rates[28]. Frequent monitoring with hourly flap checks in the first 24 h is ideal, and this frequency can be decreased to every 4 h for the subsequent 2-3 days[29]. Shorter intervals, such as 30-minute intervals for the first 24 h, are considered optimal but can be time-consuming for surgeons, residents, or nursing staff. Additionally, microsurgical flaps carry the potential for infection and vascular trauma, which, when combined with manual examination and dressing procedures, may result in the development of hematoma and seroma[30]. Nevertheless, the primary objective of any post-operative monitoring protocol should be to optimize the patient’s recovery and expedite their return to pre-operative status, thereby reducing hospital length of stay, the risk of infection or deep venous thrombosis, and costs of care[27]. Emerging research suggests that the implementation of an enhanced recovery after surgery (ERAS) program, which incorporates a transdisciplinary comprehensive approach to peri-operative care, may effectively reduce post-operative complications, shorten the length of stay, and minimize the need for morphine equivalent dosing[31]. High-volume institutions and greater provider experience have been associated with lower rates of flap failure, indicating the beneficial role of protocols[32,33]. Typically, patients are placed on strict bed rest and “nothing by mouth” for the first 24 h to prevent mechanical complications and allow for prompt re-exploration, if necessary. Volatile hemodynamic disturbances are avoided by using appropriate anesthetic/pharmacologic agents[20,34,35]. The use of anticoagulation remains controversial due to the delicate balance between undesirable bleeding and the risk of thrombosis[36]. However, prophylactic administration of heparin can be employed for deep-vein thrombosis prevention. Aspirin can be given for up to 30 days to inhibit platelet aggregation[37]. Low-molecular-weight heparin and aspirin, either alone or in combination, have shown similar efficacy in reducing macrovascular graft occlusion after surgery[36]. Lastly, patient education about the planned monitoring can help to manage their expectations, improve compliance, and enhance both flap outcomes as well as the overall patient experience[38].
Bedside clinical evaluation
The clinical exam remains the cornerstone of flap monitoring after breast reconstruction, offering high sensitivity and effectiveness in detecting failing flaps without incurring the expenses associated with advanced monitoring technologies or specialized personnel. The clinical exam comprises four essential components: color, capillary refill, tension/turgor/swelling, and temperature [Table 1][39]. Unlike software-based techniques, the clinical exam has the capability to discern between adequate inflow (arterial) and outflow (venous) problems[39]. While not all patients will have a drain post-operatively, checking the drain’s functionality, output color, and amount remains an important aspect of the clinical exam.
Clinical examination findings
Characteristics | Arterial | Venous | |
Visual | Color | Pale | Cyanotic, dusky |
Capillary Refill | Delayed (> 3 secs) | Shortened (< 2 secs) | |
Pinprick | Decreased | Dark venous outflow | |
Tactile | Temperature | Cold | Warmth |
Tension/Swelling | Mild swelling (late finding) | Increased turgor |
The effectiveness of the clinical exam relies heavily on the experience of the provider, who may assign varying importance to specific exam findings. Furthermore, patient-specific factors, such as skin tone and flap location, can influence findings; color changes, for instance, may be less pronounced in patients of different races and/or ethnicities[40,41]. The clinical exam has limitations in cases where flaps lack a cutaneous component (e.g., musculocutaneous flaps and other buried flaps). In such instances, surgeons may opt to expose a small portion of the flap at the skin surface temporarily to facilitate monitoring. Advanced monitoring technologies are particularly valuable when the clinical exam yields ambiguous or inconclusive results[42].
Adjunctive techniques
Medical devices manufacture is an evolving field and an area of ongoing research. The objective of developing flap monitoring devices is to create compact, portable techniques that can provide consistent, real-time quantitative data on flap viability and perfusion[43-47]. The data obtained should be objective and not provider-dependent[48,49]. This data can assist surgeons in assessing flow patterns, quality, severity, and determining the need for reoperation[50].
Typically, techniques for flap monitoring are categorized as either non-invasive [Tables 1 and 2] or invasive [Table 3]. Multiple devices can be used in combination as adjuncts to the clinical exam. Generally, non-invasive techniques are more user-friendly, readily available, and cost-effective. Quantitative results that can be tracked over time enhance the sensitivity of detecting flap failure. Monitoring devices may enable flap monitoring without direct contact with providers, which was advantageous during the COVID-19 pandemic[51]. However, it is important to note that absolute values may have limitations and lack consistency between patients. Further, the use of monitoring machines may entail costs and require specialized expertise[48].
Non-invasive techniques for flap monitoring including main benefits and limitations
Non-invasive technique | Benefits | Limitations |
Physical examination | ● Combines several signs (visual, tactile, drain output) to determine the risk of flap failure ● Opportunity for patient interaction to counsel, manage anxiety and concern, provide answers to questions, and participate in shared decision making post-operatively (e.g., ensuring bed rest and “nothing by mouth”) | ● Subjective to provider’s gestalt and experience ● Not quantitative ● Limited in flaps without cutaneous portion ● Lack of continuous monitoring |
Acoustic doppler ultrasonography | ● Minimal training required ● Highly portable, bedside utilization ● Cost effective ● Intra- and post-operative use ● Simple to pair with concurrent physical examination | ● Accuracy can be impacted by noise from vessels near the target vessel ● Blood flow detected is not always sufficient to sustain flap ● Unsuitable for buried flaps |
Laser doppler flowmetry | ● Intra- and post-operative flap use ● Relatively easy to interpret and can be monitored by many members of the care team ● Blood flow velocity readings are continuously reported, giving a quantitative picture of blood flow ● Accurate flow and velocity measurements in vessels up to 8 mm deep | ● Blood flow measurements are not absolute but relative, depending on specific device and patient parameters, making a definitive threshold for vascular compromise elusive ● Many of the pieces of the laser flowmeter apparatus are fragile and can be easily broken by patients and providers alike False reports of inadequate flow can be reported when a blood clot or other obstruction blocks the laser’s path |
Color duplex ultrasound | ● Improved sensitivity for detection of vascular compromise compared to other Doppler methods ● Can detect and discern discrete vascular pathologies | ● Large and unwieldy ● Requires expert operation by a technician as well as the presence of an attending microsurgeon or resident to orient the device ● Expensive to acquire and maintain ● Not as useful in the intraoperative setting |
Surface temperature monitoring with/without infrared thermal device | ● Cost effective ● Bedside ● Real-time ● Not provider dependent ● Can be non-contact ● Quantitative ● Trends can be monitored overtime | ● Limited value in isolation without combined clinical judgment (spurious results possible, e.g., if not in contact) ● Cannot be used in flaps without cutaneous portion |
Tissue oximetry | ● Bedside ● Real-time ● Not provider dependent ● Quantitative ● Trends can be monitored overtime ● Highly portable | ● Readings influenced by ambient light ● Readings influenced by patient movement ● Readings can vary by device type ● Different skin colors have been reported to produce different results ● Does not directly assess vascular patency (measures end tissue oxygenation) ● Cost |
Invasive techniques for flap monitoring, including main benefits and limitations
Invasive technique | Benefits | Limitations |
Implantable doppler & venous coupler | ● Cost effective ● Usable on all types of flaps ● Ease in signal reading | ● May cause vessel damage ● Complex operative technique ● High false positive rates |
Transcutaneous oxygen tension monitoring | ● Very sensitive to declines in tissue oxygenation ● Easy to monitor ● Can be used for monitoring of buried flaps ● Provides a direct measurement of oxygen availability at the cellular level | ● Invasive, requires tunneling with some extra dermal components ● May be unreliable in large cutaneous flaps or flaps with large within-flap temperature gradients |
Biochemical markers | ● Cost effective ● Reflect the flap’s local environment ● Patterns of known metabolites exist to direct thresholds and clinical decisions | ● Limited efficacy among diabetic patients ● Not applicable for buried flaps |
Microdialysis | ● Can be used when clinical examination is not possible ● Detects flap failure hours before it becomes clinically evident ● Gives a quantitative metric | ● Causes local tissue trauma ● Cost ● High false-positive rates ● May require an equilibrium period ● Securing the catheter can be difficult |
Fluorescence imaging | ● Broadly used with clinical applications beyond plastic surgery ● Can leverage different fluorophores ● Handheld devices and probes have been developed ● Ease of use | ● Additional staffing requirements are not as high as other techniques ● Continuous monitoring is difficult ● Repeat injections of dyes may be required ● Limited circulation of fluorophores |
Technetium-99m sestamibi scintigraphy | ● Can monitor muscle flap viability reliably ● Reveals distal ischemia or hypoperfused area in muscle tissue ● Short half-life of ~6 h | ● Limited to a majority of case reports and series with the need for more robust studies ● May need to be combined with other techniques, such as Doppler, to visualize the blood vessel integrity |
Perfusion-weighted MRI | ● Is relatively non-invasive, and risks to the patient are limited to those imposed by the administration of contrast ● Provides a picture of entire-flap perfusion | ● Resource intensive ● Requires support staff at each step ● Requires serial studies if flap is to be monitored over time |
Non-invasive techniques
Photoelectric assessment with Doppler monitoring
Doppler ultrasonography is widely recognized as one of the most effective and commonly used non-invasive methods for flap monitoring, with acoustic Doppler devices being the most predominant choice. Acoustic Dopplers operate by emitting sound waves of a specific frequency, phase, and amplitude into the tissue. These sound waves reflect off moving matter, primarily the red blood cells within the intravascular arterial and venous system in flaps. The movement of these cells leads to a slight shift in the frequency of the reflected sound waves, known as the Doppler effect, hence the term “Doppler”. The machine converts and transmits these reflections into an audible noise, which exhibits a pulsatile pattern in arterial flow and a quieter, more consistent pattern in venous flow[52-54]. Marking arterial and venous acoustic signals at the conclusion of the procedure with a single stitch can facilitate post-operative monitoring[55]. Acoustic Doppler sonography is a user-friendly technique that requires minimal training, and the devices are widely available, cost-effective, and highly portable[54]. However, it is important to note that the absence of a signal does not always indicate inadequate blood supply for flap viability, and the signal from key perforators may be difficult to distinguish from background noise caused by other vessels[53].
Laser flowmetry is another Doppler-based method employed for flap monitoring, although it differs from acoustic Doppler by measuring in light waves instead of sound waves[56]. Laser flowmetry probes are secured to the skin surface overlying the target vessel using dressings or sutures[57,58]. The data output provides real-time measurements of skin surface blood velocity and blood flow, which are accurate up to a depth of
Color duplex ultrasound is a less commonly employed Doppler-based method for non-invasive flap monitoring, utilizing a device that emits and detects the entire visual spectrum of light[60]. The device detects the Doppler effect, which is the change in frequency between the emitted and reflected visible light waves. These light waves are transduced to create a grayscale ultrasound image that is augmented with color to indicate the direction and position of blood flow. Color duplex ultrasound has proven successful in monitoring various flaps with different vessel sizes, including fasciocutaneous perforators for DIEP flaps[61]. A variant device known as “power Doppler imaging” uses higher frequency waves to detect vessels with smaller diameters, reaching as low as 0.2 mm[62]. Color Doppler ultrasound is highly sensitive in detecting compromised vessels, enabling the assessment of vessel caliber, patency, flow characteristics, anomalies, and pathologies[54]. However, its specificity in identifying vascular compromise, like other Doppler devices, is limited. Color duplex ultrasound machines are more expensive, less portable, and require trained personnel for operation. Typically, the presence of a microsurgeon is necessary to aid in the anatomical orientation of the transmitter, while a radiology technician is required for consistent image acquisition and interpretation.
Surface temperature monitoring
In addition to tactile assessment in the clinical exam, flap temperature can be measured and monitored using temperature probes placed on the skin surface. The arterial blood supplying the flap carries heat, which is released into the surrounding extravascular tissue through convection[63]. Congested flaps exhibit distinct temperature drop patterns compared to ischemic flaps. A temperature drop of
Recently, infrared thermography has emerged as a novel method of post-operative flap monitoring in breast reconstruction[67,68]. This thermal imaging technology converts the detected temperature values through infrared radiation into pixel values, resulting in a visual display of cutaneous blood flow. Infrared thermography has demonstrated a sensitivity of 96% and a specificity of 75%, indicating its potential as a valuable adjunct to clinical assessment[67].
Surface temperature monitoring has its limitations when applied to buried and intraoral flaps[69], and it can be influenced by various external factors, including ambient room temperature and air flow. Research suggests that in patients who have undergone DIEP breast reconstruction, surface temperature monitoring using a dual-channel digital thermometer (Raytek, Norway) lacks sensitivity and is inferior to clinical examination conducted by trained nurses[70]. In the case of large perforator flaps such as DIEPs, the temperature difference between the flap surface and adjacent non-flap surface may be minimal, reducing the utility of surface temperature monitoring. However, it may still have value in monitoring perfusion in scenarios like digit replantation and small free flap reconstruction[71].
Mobile smartphone monitoring technology
Given the widespread use of smartphones, leveraging this technology to enhance communication and enable remote post-operative monitoring through adjunct devices presents a rapid, cost-effective, and non-invasive option for early detection of free flap failure.
Innovative text messaging systems that alert surgical teams of post-operative monitoring tissue oximetry readings below a certain threshold show promise in identifying potential flap loss and facilitating prompt notification[72]. With smartphones equipped with advanced digital photography capabilities, earlier discharge and continued remote flap monitoring at home with improved communication between patients and healthcare staff may soon become possible. Studies suggest that the use of remote digital photography may even lead to reduced response time to re-exploration and improved flap salvage rates[39,73]. The usefulness of digital photography by patients becomes even more apparent when uploaded through dedicated post-operative monitoring platforms, enabling easy comparison with previous images[74]. By employing free applications on smartphones, continuous real-time streaming of free flap tissue to surgeons for decision-making can be achieved[75]. Kiranantawat et al. developed a smartphone application that identifies color changes indicative of compromised flaps for monitoring purposes[76]. Furthermore, the integration of machine learning algorithms holds the potential to reveal previously undetectable findings to the human eye[77].
Digital thermographic cameras, such as the FLIR ONE ™ device (FLIR systems, Wilsonville, Ore), can be attached to a smartphone to capture thermal images of a free flap, enabling the detection of temperature differences with high sensitivity. These images can be easily transmitted to healthcare providers for evaluation and early identification of flap failure. While this device has primarily been used by trained operators in the peri-operative setting for DIEP flap reconstruction, advancements in technology and reduced costs hold the potential for future remote use by patients[78]. However, it is important to note that this device is influenced by external factors such as vasoconstriction, warming blankets, or low ambient temperature, which may impact its accuracy.
Tissue oximetry
Tissue oximetry utilizes both near-infrared spectroscopy (NIRS) (wavelength: 800-2,500 nanometers) and visible light (wavelength: 40-700 nanometers) to non-invasively measure flap perfusion[79-83]. Examples of tissue oximeters commonly used in the post-operative setting include Spectros T-Stat (Houston, TX, USA) and ViOptix T.Ox (Newark, CA, USA) [Figure 1A]. The functioning principle of most oximetry systems follows a standard pattern: the oximeter devices emit light towards the flap tissue, a portion of which is absorbed by the blood. The remaining light is reflected and captured by the sensors in the oximeter. Oxygenated and deoxygenated blood reflect and emit light differently, and the oximeter probes utilize these properties to estimate the percentage of oxygenated and deoxygenated hemoglobin within the underlying tissue[13,69]. A decrease in tissue oxygenation detected by NIRS has shown high specificity and sensitivity in detecting flap failure[84]. However, conflicting reports regarding time-based trends in post-operative flap oximetry exist, with some observing transient decreases, increases, or stable flap oxygenation. These trends may be attributed to fluctuations in hemodynamic status (i.e., pulse oximetry) rather than perfusion status[85].
Figure 1. Types of non-invasive and invasive techniques used for flap monitoring. (A): Example of a non-invasive tissue oximetry device; (B): Example of an invasive venous coupler and implantable Doppler monitoring device; (C): Example of an invasive transcutaneous oxygen tension monitoring device.
Tissue oximetry devices serve as valuable adjuncts for flap monitoring due to their high portability, user-friendliness, and ability to provide continuous monitoring. Rather than focusing on absolute values, observing the individual oximetry trend of a flap provides greater insight into perfusion. It is important to note that while oximetry indicates organ tissue oxygenation, which directly reflects flap viability as opposed to vascular patency[80], there can be delays in detection of up to 1-5 h[86]. Additionally, environmental light and variations in skin tones can potentially interfere with accurate readings[40-41,87]. In a recent study involving over 1,000 free flaps for breast reconstruction, tissue oximetry offered no benefit over clinical judgment, although other reports present contradictory evidence[88,89]. Bai et al. conducted experiments using porcine models to test the use of NIRS in flaps without cutaneous paddles. NIRS probes were buried into muscle tissue to provide continuous monitoring of local tissue oxygen saturation, demonstrating the capability of heat convection probes to detect changes in tissue microcirculatory blood flow[43,90].
Implementation of tissue oximetry for routine monitoring incurs additional costs, with equipment expenses amounting to $16,500 and disposable sensor costs of $150 per unit[13]. These initial costs must be weighed against the estimated savings, which have been reported to reach as high as $1,667 with the utilization of NIRS monitoring protocols in DIEP flap reconstruction[91].
Invasive techniques
Invasive techniques for free flap monitoring are utilized for buried flaps when surface monitoring is not feasible. An ideal implantable device should possess the following characteristics: (1) enable continuous real-time monitoring of anastomotic patency; (2) reliably distinguish between arterial and venous occlusions; and (3) be applicable for monitoring both cutaneous and buried flaps. Various devices have been developed for invasive flap monitoring and ongoing research focuses on device refinement[42-47]. Current electronic flap monitoring techniques offer qualitative and quantitative information on flap perfusion, including flow patterns and quality[48-50]. However, there are limitations associated with the use of invasive monitoring techniques, such as the requirement for expert interpretation of results, inadequate equipment designs and potential malfunction, and high costs[48].
Implantable Doppler & venous coupler
Swartz et al. were the first to publish on the utilization of an implantable probe for continuous monitoring of microvascular anastomoses[92]. Their study employed a 20-MHz ultrasonic Doppler probe encased in a silicone sleeve positioned distal to an anastomosis, demonstrating the probe’s effectiveness in monitoring patency and occlusion up to 4 weeks post-operatively[92]. This system, now referred to as the Cook-Swartz implantable Doppler (Cook Medical®, Ireland), has since become a valuable tool in clinical post-operative monitoring, particularly for buried flaps, such as those in nipple-sparing mastectomy procedures [Figure 1B]. Apart from the Cook-Swartz implantable Doppler used in buried flaps, an external transcutaneous Doppler signal can be marked on the mastectomy skin intra-operatively, following temporary clamping of the flap vascular pedicle, to minimize potential signals confusion between the mastectomy skin and the flap. In 1994, Swartz et al. discovered that Cook monitoring of venous anastomoses exhibited greater sensitivity to both venous and arterial thrombosis compared to solely detecting arterial anastomoses[92,93]. Since its introduction, there have been numerous advancements aimed at enhancing the monitoring of capabilities of Cook Doppler devices, which heavily rely on their positioning. These improvements encompass non-attachment around a venous pedicle, microclip fixation, suture fixation, and elongation of the silicone cuff. The success of these developments has varied, and their implementation may lead to prolonged operating times and necessitate technical expertise to ensure proper intraoperative placement[94,95]. Additionally, complications associated with Cook Dopplers include retained wires, pedicle laceration during extraction, and signal interruption due to clot formation around the probes[96].
The Flow Coupler is an additional invasive post-operative monitoring device designed to detect the patency of microsurgical anastomoses [Figure 1B]. Developed by Synovis Life Technologies in 2010, this device consists of a removable 20-MHz Doppler that provides real-time data on vessel patency. Preliminary data suggests that the Flow Coupler is both effective and reliable. However, studies comparing the results of the Cook-Swartz implantable Doppler and the Synovis Flow Coupler have not identified any significant differences in false positive or false negative results, or the need for salvage procedures[97]. The Flow Coupler may have a higher false positive rate and a higher incidence of vascular thrombotic events compared to non-flow coupler devices[98]. A pooled meta-analysis revealed that the use of a Cook-Swartz Doppler in combination with clinical monitoring is associated with significantly higher rates of flap survival but also increased Incidence of false positive results[96]. When compared to microdialysis, the implantable Cook-Swartz Doppler demonstrated earlier detection of flap compromise by 60 min and reduced time to re-exploration compared to external Doppler monitoring[99,100].
Transcutaneous oxygen tension monitoring
Tissue oxygen tension monitoring is a commonly used invasive method to assess flap perfusion and viability [Figure 1C][101]. This technique involves the use of probes that compare the partial pressure gradient between oxygen delivered at the capillary level and mitochondrial consumption, providing a direct estimation of oxygen availability at the cellular level[102,103]. To achieve tissue oxygen tension monitoring, an implantable probe and microcatheter are typically used, with the microcatheter containing an electrode functioning as an oxygen sensor[104]. When the apparatus is placed in the flap, it measures the reduction of molecular oxygen using a cathode and polarized circuit[101,105]. Rapid declines in oxygen tension detected by these sensors indicate potential vascular compromise[106].
Oxygen tension monitoring devices offer real-time measurements of oxygenation, allowing for trend analysis over time. These measurements are easily understandable by all members of the care team and are considered highly sensitive indicators of end-organ tissue perfusion[107-109]. However, these devices require invasive procedures for placement and removal of probes, which carries the risk of flap infection. Furthermore, the temperature calibration of probes makes them susceptible to the influence of external environmental factors and subtle temperature variations within the flap. Consequently, some authors have suggested that these systems may be more prone to bias and could benefit from the integration of other flap monitoring approaches, particularly in cases involving large areas of skin or significant temperature gradients within the flap[101,110].
Fluorescence imaging
Fluorescein angiography was one of the early applications of fluorescence in measuring flap perfusion[111]. Depending on the specific use case, different fluorophores can be leveraged to monitor perfusion quality and flap viability. Indocyanine green fluorescence angiography (ICG-FA) has been extensively used for peri-operative assessment of anastomotic patency and has found applications in various fields, such as cardiac function monitoring, liver function testing, neurosurgery, and ophthalmology. As a non-toxic, water-soluble dye with a half-life of 3-4 min, ICG can be safely injected into patients to visualize microvascular patency and tissue perfusion. Adelsberger et al. conducted a study involving 210 free flaps to assess vascular thromboses post-operatively. They used a handheld infrared camera in a dark room to visualize the distribution of the dye after injection over a 4-hour interval for the first 72 h[112]. The combination of ICG-FA and clinical examination yielded an 85% success rate in detecting vascular thromboses. Revision rates decreased from 19% to 12%, with a false negative revision rate of 4.8% over a span of 3 years, accounting for a training and habituation period. Others have combined ICG injection with scanning of flap using a near-infrared camera with an additional pinprick test to evaluate perfusion status by fluorescent imaging of bleeding. This approach facilitates the need for repeated pinpricks and may lead to subsequent complications, such as hematoma. However, it is particularly useful in patients with questionable perfusion[113]. Hitier et al. determined that intraoperative fluorescence after anastomosis to the recipient vessels serves as a reliable predictor of post-operative flap viability, capable of indicating abnormal values 16 hours prior to clinical evidence of flap failure using fluorescence signal thresholds[114]. Despite its usefulness, ICG-FA has certain limitations. It does not allow for continuous monitoring and requires repeat injections. Exploring other fluorophores with increased circulation time may enable continuous monitoring and provide activation at different wavelengths.
Other monitoring techniques
Given the significant advancements in post-operative monitoring techniques for autologous breast reconstruction proven to have a high sensitivity and specificity, there has been a shift away from more outdated and costly monitoring techniques. These include the use of biochemical markers, microdialysis, technetium-99m sestamibi scintigraphy, and perfusion-weighted MRI. While we include them here for a comprehensive perspective, it is important to note their limitations in terms of invasiveness, cost, and lack of continuous monitoring.
Biochemical markers
Using a femoral vessel anastomosis rodent model, Su et al. reported in 1982 that both venous and arterial occlusion decreased tissue glucose content and increased lactate content. Venous occlusion was found to have a more detrimental effect on flap survival[115]. The theory behind this observation suggests that vessel occlusion impairs perfusion, resulting in decreased tissue oxygenation and glucose delivery, leading to a shift from aerobic to anaerobic metabolism[116-118].
Since then, tissue biomarkers such as glucose with or without lactate have been successfully used for flap monitoring in the clinical setting[119-122]. These biomarkers have been utilized at different threshold values and rates of change to indicate venous occlusion, showing variable sensitivity and specificity. Measurement of flap tissue glucose levels is a simple, inexpensive, and rapid option for post-operative flap monitoring compared to other techniques[13]. However, the efficacy of glucose measurements in detecting arterial occlusion and in diabetic patients is limited, and this technique lacks applicability in buried flaps[123-125].
Microdialysis
Microdialysis has been employed as a method for microinvasive monitoring of flap ischemia in various flap types, including myocutaneous, buried flaps and TRAM flaps[126-129]. This technique involves a double-lumen catheter with a semipermeable membrane at the end, which draws fluid from the flap through the membrane into the catheter. By continuously assessing small molecules in the dialysate, such as glucose, lactate, pyruvate, and glycerol, microdialysis provides a measure of the flap’s metabolic activity. These values can be compared values to a baseline value or ratios, non-flap tissue away from the operative site, or reference values. The analysis of the interstitial fluid contents allows for flap examination without the need for direct clinical examination and can potentially detect ischemia one to two hours before clinical evidence of flap failure[130]. This technique is limited by its high cost and relatively high false-positive rate. Additionally, widespread adoption is restricted by the requirement for a skilled nursing team and clinical familiarity with microdialysis.
Technetium-99m sestamibi scintigraphy
Technetium-99m (99mTc) sestamibi, a metabolically inactive radionuclide, is injected and measured by scintigraphy to visualize areas of tissue hypoperfusion and evaluate microvascular anastomotic patency. It has been employed to assess free muscle flap viability, particularly in detecting a delayed secondary thrombosis caused by microemboli, which may not be evident using other techniques relying on more obvious signs[131-133]. Additionally, this technique permits visualization of partial necrosis, providing important information of surgical significance in the case of future surgical debridement[131].
Perfusion-weighted MRI
Several reports have described the use of contrast-enhanced magnetic resonance imaging (MRI) as a method for analyzing flap perfusion, with parameters optimized for vascular contrast uptake[134,135]. Compromised flaps exhibit significantly reduced signal intensity on MRI[134,135]. Perfusion-weighted MRI can assess the overall perfusion of the entire free flap. The drawbacks of this technique are mostly resource-related: the financial and time-based costs of MRIs are high. Additionally, perfusion-weighted MRI does not directly evaluate vascular pathology and necessitates specialized support staff throughout the process.
Future directions
In the past 40 years, post-operative flap monitoring technologies have advanced to provide continuous, remote, highly sensitive, and non-invasive options for surgeons. Lee et al. developed a new dual-camera flap monitoring system that combines mask region-based convolutional neural networks in the visible-light system with infrared systems to address lower image resolution challenges posed by illumination changes and patient movement[136,137]. This study demonstrated earlier detection of vascular congestion compared to manual observation. Kim et al. utilized a novel negative pressure wound therapy technique using a transparent film to monitor the entire flap, allowing for the examination of flap color changes, capillary refilling status, and flap warmth without missing any portions of the flap[30]. This technique has been shown to reduce monitoring time and costs[30]. Videocapillaroscopy technology shows further promise in evaluating circulatory changes on the skin surfaces of free flaps while deliberately clamping pedicle vessels[138]. Bucknor et al. described a novel, non-invasive, optical oxygen-sensing liquid bandage for post-operative monitoring in autologous breast reconstruction[139]. This technique uses a camera to continuously capture the intensity of oxygen phosphorescence and fluorescence. Hummelink et al. used a “free flap patch” adherent to a flap to continuously measure temperature and tissue saturation[140]. The development of novel, patient-friendly wearable wireless monitors is ongoing, promising rapid and real-time insights into tissue perfusion.
CONCLUSION
Careful post-operative monitoring of free flaps following breast reconstructive surgery is crucial for determining procedural success. While the clinical examination remains the gold standard for post-operative flap monitoring, various adjuncts exist to complement and enhance our understanding of flap perfusion.
DECLARATIONS
Authors’ contributionsMade substantial contributions to the conception and design of the study and performed data analysis and interpretation: Cevallos PC, Najafali D, Johnstone TM
Performed data acquisition, as well as provided administrative, technical, and material support: Borrelli MR, Manrique OJ, Lee GK, Nazerali RS
Availability of data and materialsNot applicable.
Financial support and sponsorshipNone.
Conflicts of interestAll authors declared that there are no conflicts of interest.
Ethical approval and consent to participateNot applicable.
Consent for publicationNot applicable.
Copyright© The Author(s) 2023
REFERENCES
1. Saber AY, Hohman HM, Dreyer MA. Basic flap design. Available from: https://www.ncbi.nlm.nih.gov/books/NBK563252/ [Last accessed on 19 Jun 2023].
2. Wolff KD. New aspects in free flap surgery: Mini-perforator flaps and extracorporeal flap perfusion. J Stomatol Oral Maxillofac Surg 2017;118:238-41.
3. Cen H, Jin R, Yu M, Weng T. Clinical decision model for the reconstruction of 175 cases of scalp avulsion/defect. Am J Otolaryngol 2021;42:102752.
4. Neusner AD, Pribaz JJ, Guo L. Free your mind, not your flap. Plast Reconstr Surg Glob Open 2022;10:e4384.
5. Wong AK, Joanna Nguyen T, Peric M, et al. Analysis of risk factors associated with microvascular free flap failure using a multi-institutional database. Microsurgery 2015;35:6-12.
6. Crawley MB, Sweeny L, Ravipati P, et al. Factors Associated with free flap failures in head and neck reconstruction. Otolaryngol Head Neck Surg 2019;161:598-604.
7. Kalmar CL, Drolet BC, Kassis S, Thayer WP, Higdon KK, Perdikis G. Breast reconstruction free flap failure: does platelet count matter? Ann Plast Surg 2022;89:523-8.
8. Novakovic D, Patel RS, Goldstein DP, Gullane PJ. Salvage of failed free flaps used in head and neck reconstruction. Head Neck Oncol 2009;1:33.
9. Sharp O, Masud D. Breast reconstruction with immediate autologous free tissue transfer in a peri-operative COVID-19 positive patient: a case report illustrating feasibility of aftercare. J Plast Reconstr Aesthet Surg 2021;74:644-710.
10. Billig JI, Lu Y, Momoh AO, Chung KC. A nationwide analysis of cost variation for autologous free flap breast reconstruction. JAMA Surg 2017;152:1039-47.
11. Dassonville O, Bozec A, Château Y, et al. Multicenter prospective micro-costing study evaluating mandibular free-flap reconstruction. Eur Arch Otorhinolaryngol 2017;274:1103-11.
12. Vonlanthen R, Slankamenac K, Breitenstein S, et al. The impact of complications on costs of major surgical procedures: a cost analysis of 1,200 patients. Ann Surg 2011;254:907-13.
13. Smit JM, Zeebregts CJ, Acosta R, Werker PMN. Advancements in free flap monitoring in the last decade: a critical review. Plast Reconstr Surg 2010;125:177-85.
14. Hauge EM, Balling E, Hartmund T, Hjortdal VE. Secondary ischemia caused by venous or arterial occlusion shows differential effects on myocutaneous island flap survival and muscle ATP levels. Plast Reconstr Surg 1997;99:825-33.
15. Kerrigan CL, Wizman P, Hjortdal VE, Sampalis J. Global flap ischemia: a comparison of arterial versus venous etiology. Plast Reconstr Surg 1994;93:1485-97.
16. Kwok AC, Agarwal JP. An analysis of free flap failure using the ACS NSQIP database. does flap site and flap type matter? Microsurgery 2017;37:531-8.
17. Kroll SS, Schusterman MA, Reece GP, et al. Timing of pedicle thrombosis and flap loss after free-tissue transfer. Plast Reconstr Surg 1996;98:1230-3.
18. Wang W, Ong A, Vincent AG, Shokri T, Scott B, Ducic Y. Flap failure and salvage in head and neck reconstruction. Semin Plast Surg 2020;34:314-20.
19. Yang Q, Ren ZH, Chickooree D, et al. The effect of early detection of anterolateral thigh free flap crisis on the salvage success rate, based on 10 years of experience and 1072 flaps. Int J Oral Maxillofac Surg 2014;43:1059-63.
20. Brown JS, Devine JC, Magennis P, Sillifant P, Rogers SN, Vaughan ED. Factors that influence the outcome of salvage in free tissue transfer. Br J Oral Maxillofac Surg 2003;41:16-20.
21. Liu EH, Zhu SL, Hu J, Wong N, Farrokhyar F, Thoma A. Intraoperative SPY reduces post-mastectomy skin flap complications: a systematic review and meta-analysis. Plast Reconstr Surg Glob Open 2019;7:e2060.
22. Hirigoyen MB, Urken ML, Weinberg H. Free flap monitoring: a review of current practice. Microsurgery 1995;16:723-7.
23. Hidalgo DA, Disa JJ, Cordeiro PG, Hu QY. A review of 716 consecutive free flaps for oncologic surgical defects: refinement in donor-site selection and technique. Plast Reconstr Surg 1998;102:722-34.
24. Okazaki M, Asato H, Takushima A, et al. Analysis of salvage treatments following the failure of free flap transfer caused by vascular thrombosis in reconstruction for head and neck cancer. Plast Reconstr Surg 2007;119:1223-32.
25. Kroll SS, Schusterman MA, Reece GP, et al. Choice of flap and incidence of free flap success. Plast Reconstr Surg 1996;98:459-63.
26. Baltodano P, Schalet GN, Aliu O, et al. Abstract P1. A national data-driven approach to optimizing monitoring of autologous breast free flap reconstruction: analysis of 3,666 patients. Plast Reconstr Surg Glob Open 2017;5:1.
27. Martinez CA, Boutros SG. Outpatient microsurgical breast reconstruction. Plast Reconstr Surg Glob Open 2020;8:e3109.
28. Mashrah MA, Aldhohrah T, Abdelrehem A, et al. Postoperative care in ICU versus non-ICU after head and neck free-flap surgery: a systematic review and meta-analysis. BMJ Open 2022;12:e053667.
29. Abouyared M, Katz AP, Ein L, et al. Controversies in free tissue transfer for head and neck cancer: a review of the literature. Head Neck 2019;41:3457-63.
30. Kim TH, Park JH. A novel negative pressure wound therapy (NPWT) monitoring system for postoperative flap management. Medicine 2021;100:e27671.
31. Tan YZ, Lu X, Luo J, et al. Enhanced recovery after surgery for breast reconstruction: pooled meta-analysis of 10 observational studies involving 1,838 patients. Front Oncol 2019;9:675.
32. Koolen PGL, Vargas CR, Ho OA, et al. Does increased experience with tissue oximetry monitoring in microsurgical breast reconstruction lead to decreased flap loss? the learning effect. Plast Reconstr Surg 2016;137:1093-101.
33. Kohler LH, Köhler H, Kohler S, et al. Hyperspectral imaging (HSI) as a new diagnostic tool in free flap monitoring for soft tissue reconstruction: a proof of concept study. BMC Surg 2021;21:222.
34. Setala L, Gudaviciene D. Glucose and lactate metabolism in well-perfused and compromised microvascular flaps. J Reconstr Microsurg 2013;29:505-10.
35. Bui DT, Cordeiro PG, Hu QY, Disa JJ, Pusic A, Mehrara BJ. Free flap reexploration: indications, treatment, and outcomes in 1193 free flaps. Plast Reconstr Surg 2007;119:2092-100.
36. Salgado CJ, Chim H, Schoenoff S, Mardini S. Postoperative care and monitoring of the reconstructed head and neck patient. Semin Plast Surg 2010;24:281-7.
37. Becattini C, Agnelli G. Aspirin for prevention and treatment of venous thromboembolism. Blood Rev 2014;28:103-8.
38. Nazir H, Lowe D, Rogers SN. Patients’ experience of the monitoring of free flaps after reconstruction for oral cancer. Br J Oral Maxillofac Surg 2017;55:1008-12.
39. Hwang J, Mun GH. An evolution of communication in postoperative free flap monitoring: using a smartphone and mobile messenger application. Plast Reconstr Surg 2012;130:125-9.
40. Bickler PE, Feiner JR, Severinghaus JW. Effects of skin pigmentation on pulse oximeter accuracy at low saturation. Anesthesiology 2005;102:715-9.
41. Feiner JR, Severinghaus JW, Bickler PE. Dark skin decreases the accuracy of pulse oximeters at low oxygen saturation: the effects of oximeter probe type and gender. Anesth Analg 2007;105:S18-23.
42. Rosenberg JJ, Fornage BD, Chevray PM. Monitoring buried free flaps: limitations of the implantable doppler and use of color duplex sonography as a confirmatory test. Plast Reconstr Surg 2006;118:109-15.
43. Bai W, Guo H, Ouyang W, et al. Intramuscular near-infrared spectroscopy for muscle flap monitoring in a porcine model. J Reconstr Microsurg 2022;38:321-7.
44. Thiem DGE, Römer P, Blatt S, Al-Nawas B, Kämmerer PW. New approach to the old challenge of free flap monitoring-hyperspectral imaging outperforms clinical assessment by earlier detection of perfusion failure. J Pers Med 2021;11:1101.
45. Halani SH, Hembd AS, Li X, et al. Flap monitoring using transcutaneous oxygen or carbon dioxide measurements. J Hand Microsurg 2022;14:10-8.
46. Wu C, Rwei AY, Lee JY, et al. A wireless near-infrared spectroscopy device for flap monitoring: proof of concept in a porcine musculocutaneous flap model. J Reconstr Microsurg 2022;38:96-105.
47. Lartizien R, Planat-Chrétien A, Berger M, et al. Noninvasive monitoring of deep tissue oxygenation in buried flaps by time-resolved near-infrared spectroscopy in pigs. Plast Reconstr Surg 2020;146:565e-77e.
48. Kwasnicki RM, Noakes AJ, Banhidy N, Hettiaratchy S. Quantifying the limitations of clinical and technology-based flap monitoring strategies using a systematic thematic analysis. Plast Reconstr Surg Glob Open 2021;9:e3663.
49. Gurtner GC, Jones GE, Neligan PC, et al. Intraoperative laser angiography using the SPY system: review of the literature and recommendations for use. Ann Surg Innov Res 2013;7:1.
50. Heller L, Levin LS, Klitzman B. Laser doppler flowmeter monitoring of free-tissue transfers: blood flow in normal and complicated cases. Plast Reconstr Surg 2001;107:1739-45.
51. Bulstrode NW, Wilson GR, Inglis MS. No-touch free-flap temperature monitoring. Br J Plast Surg 2002;55:174.
52. Hallock GG. Attributes and shortcomings of acoustic doppler sonography in identifying perforators for flaps from the lower extremity. J Reconstr Microsurg 2009;25:377-81.
53. Hallock GG. Doppler sonography and color duplex imaging for planning a perforator flap. Clin Plast Surg 2003;30:347-57.
54. Hallock GG. Acoustic Doppler sonography, color duplex ultrasound, and laser doppler flowmetry as tools for successful autologous breast reconstruction. Clin Plast Surg 2011;38:203-11.
55. Knobloch K, Gohritz A, Reuss E, Redeker J, Spies M, Vogt PM. Preoperative perforator imaging in reconstructive plastic surgery: current practice in Germany. Plast Reconstr Surg 2009;124:183e-4e.
56. Micheels J, Alsbjorn B, Sorensen B. Laser doppler flowmetry. a new non-invasive measurement of microcirculation in intensive care? Resuscitation 1984;12:31-9.
57. Clinton MS, Sepka RS, Bristol D, et al. Establishment of normal ranges of laser Doppler blood flow in autologous tissue transplants. Plast Reconstr Surg 1991;87:299-309.
58. Jenkins SD, Sepka RS, Barwick WJ, Serafin D, Klitzman B. Routine clinical use of laser Doppler flowmeter to monitor free tissue transfer: preliminary results. J Reconstr Microsurg 1987;3:281-3.
59. Luck JC, Kunselman AR, Herr MD, Blaha CA, Sinoway LI, Cui J. Multiple laser doppler flowmetry probes increase the reproducibility of skin blood flow measurements. Front Physiol 2022;13:876633.
60. Hallock GG. Color duplex imaging for identifying perforators prior to pretransfer expansion of fasciocutaneous free flaps. Ann Plast Surg 1994;32:595-601.
61. Hong JP, Hur J, Kim HB, Park CJ, Suh HP. The use of color duplex ultrasound for local perforator flaps in the extremity. J Reconstr Microsurg 2022;38:233-7.
62. Schwabegger AH, Bodner G, Rieger M, Jaschke WR, Ninković MM. Internal mammary vessels as a model for power doppler imaging of recipient vessels in microsurgery. Plast Reconstr Surg 1999;104:1656-65.
63. Khouri RK, Shaw WW. Monitoring of free flaps with surface-temperature recordings: is it reliable? Plast Reconstr Surg 1992;89:495-502.
64. Kraemer R, Lorenzen J, Knobloch K, et al. Free flap microcirculatory monitoring correlates to free flap temperature assessment. J Plast Reconstr Aesthet Surg 2011;64:1353-8.
65. Papillion P, Wong L, Waldrop J, et al. Infrared surface temperature monitoring in the postoperative management of free tissue transfers. Can J Plast Surg 2009;17:97-101.
66. Chiu ES, Altman A, Allen RJ Jr, Allen RJ Sr. Free flap monitoring using skin temperature strip indicators: adjunct to clinical examination. Plast Reconstr Surg 2008;122:144e-5e.
67. Rabbani MJ, Bhatti AZ, Shahzad A. Flap monitoring using thermal imaging camera: a contactless method. J Coll Physicians Surg Pak 2021;30:703-6.
68. Chang K, Yoon S, Sheth N, et al. Rapid
69. Salgado CJ, Moran SL, Mardini S. Flap monitoring and patient management. Plast Reconstr Surg 2009;124:e295-302.
71. Dam H, Nduka C, Carver N. No touch free-flap temperature monitoring. Br J Plast Surg 2003;56:835.
72. Ricci JA, Vargas CR, Lin SJ, Tobias AM, Taghinia AH, Lee BT. A novel free flap monitoring system using tissue oximetry with text message alerts. J Reconstr Microsurg 2016;32:415-20.
73. Engel H, Huang JJ, Tsao CK, et al. Remote real-time monitoring of free flaps via smartphone photography and 3G wireless internet: a prospective study evidencing diagnostic accuracy. Microsurgery 2011;31:589-95.
74. Semple JL, Sharpe S, Murnaghan ML, Theodoropoulos J, Metcalfe KA. Using a mobile app for monitoring post-operative quality of recovery of patients at home: a feasibility study. JMIR Mhealth Uhealth 2015;3:e18.
75. Yuen JC. Enabling Remote monitoring using free apps and smart devices for a free-flap adjunct monitor. Plast Reconstr Surg Glob Open 2017;5:e1507.
76. Kiranantawat K, Sitpahul N, Taeprasartsit P, et al. The first smartphone application for microsurgery monitoring: silpaRamanitor. Plast Reconstr Surg 2014;134:130-9.
77. Provenzano D, Chandawarkar A, Caterson E. Abstract QS14: novel smartphone-based free flap monitoring tool using machine learning. Plast Reconstr Surg Glob Open 2019;7:111-2.
78. Phillips CJ, Barron MR, Kuckelman J, et al. Mobile smartphone thermal imaging characterization and identification of microvascular flow insufficiencies in deep inferior epigastric artery perforator free flaps. J Surg Res 2021;261:394-9.
79. Zaman T, Kyriacou PA, Pal SK. Free flap pulse oximetry utilizing reflectance photoplethysmography. Annu Int Conf IEEE Eng Med Biol Soc 2013;2013:4046-9.
80. Keller A. Noninvasive tissue oximetry for flap monitoring: an initial study. J Reconstr Microsurg 2007;23:189-97.
81. Menick FJ. The pulse oximeter in free muscle flap surgery. “a microvascular surgeon's sleep aid”. J Reconstr Microsurg 1988;4:331-4.
82. Hallock GG, Rice DC. A comparison of pulse oximetry and laser doppler flowmetry in monitoring sequential vascular occlusion in a rabbit ear model. Can J Plast Surg 2003;11:11-4.
83. Lin SJ, Nguyen MD, Chen C, et al. Tissue oximetry monitoring in microsurgical breast reconstruction decreases flap loss and improves rate of flap salvage. Plast Reconstr Surg 2011;127:1080-5.
84. Lohman RF, Langevin CJ, Bozkurt M, Kundu N, Djohan R. A prospective analysis of free flap monitoring techniques: physical examination, external Doppler, implantable Doppler, and tissue oximetry. J Reconstr Microsurg 2013;29:51-6.
85. Ozturk CN, Ozturk C, Ledinh W, et al. Variables affecting postoperative tissue perfusion monitoring in free flap breast reconstruction. Microsurgery 2015;35:123-8.
86. Keller A. A new diagnostic algorithm for early prediction of vascular compromise in 208 microsurgical flaps using tissue oxygen saturation measurements. Ann Plast Surg 2009;62:538-43.
87. Kovalenko B, Roskosky B, Freedman BA, Shuler MS. Effect of ambient light on near infrared spectroscopy. Available from: https://www.semanticscholar.org/paper/Effect-of-Ambient-Light-on-Near-Infrared-Kovalenko-Roskosky/b8c2924e0008466beb67d41b18af5809aad9ef77. [Last accessed on 25 Jul 2023].
88. Lindelauf AAMA, Vranken NPA, Rutjens VGH, et al. Economic analysis of noninvasive tissue oximetry for postoperative monitoring of deep inferior epigastric perforator flap breast reconstruction: a review. Surg Innov 2020;27:534-42.
89. Johnson BM, Cullom ME, Egan KG, et al. Comparing tissue oximetry to doppler monitoring in 1,367 consecutive breast free flaps. Microsurgery 2023;43:57-62.
90. Lu D, Moritz W, Arafa HM, et al. Intramuscular microvascular flow sensing for flap monitoring in a porcine model of arterial and venous occlusion. J Reconstr Microsurg 2023;39:231-7.
91. Ricci JA, Vargas CR, Ho OA, Lin SJ, Tobias AM, Lee BT. Evaluating the use of tissue oximetry to decrease intensive unit monitoring for free flap breast reconstruction. Ann Plast Surg 2017;79:42-6.
92. Swartz WM JN, Cherup L, Klein A. Direct monitoring of microvascular anastomoses with the 20-MHZ ultrasonic doppler probe: an experimental and clinical study. Plast Reconstr Surg 1988;81:149-58.
93. Chadwick SL, Khaw R, Duncan J, Wilson SW, Highton L, O’Ceallaigh S. The use of venous anastomotic flow couplers to monitor buried free DIEP flap reconstructions following nipple-sparing mastectomy. JPRAS Open 2020;23:50-4.
94. Smit JM, Whitaker IS, Liss AG, Audolfsson T, Kildal M, Acosta R. Post operative monitoring of microvascular breast reconstructions using the implantable cook-swartz doppler system: a study of 145 probes & technical discussion. J Plast Reconstr Aesthet Surg 2009;62:1286-92.
95. Rozen WM, Ang GG, McDonald AH, et al. Sutured attachment of the implantable doppler probe cuff for large or complex pedicles in free tissue transfer. J Reconstr Microsurg 2011;27:99-102.
96. Hayler R, Low TH, Fung K, Nichols AC, MacNeil SD, Yoo J. Implantable doppler ultrasound monitoring in head and neck free flaps: balancing the pros and cons. Laryngoscope 2021;131:E1854-9.
97. Um GT, Chang J, Louie O, et al. Implantable cook-swartz doppler probe versus Synovis flow coupler for the post-operative monitoring of free flap breast reconstruction. J Plast Reconstr Aesthet Surg 2014;67:960-6.
98. Kempton SJ, Poore SO, Chen JT, Afifi AM. Free flap monitoring using an implantable anastomotic venous flow coupler: analysis of 119 consecutive abdominal-based free flaps for breast reconstruction. Microsurgery 2015;35:337-44.
99. Frost MW, Niumsawatt V, Rozen WM, Eschen GE, Damsgaard TE, Kiil BJ. Direct comparison of postoperative monitoring of free flaps with microdialysis, implantable cook-swartz doppler probe, and clinical monitoring in 20 consecutive patients. Microsurgery 2015;35:262-71.
100. Hosein RC, Cornejo A, Wang HT. Postoperative monitoring of free flap reconstruction: a comparison of external doppler ultrasonography and the implantable doppler probe. Plast Surg 2016;24:11-9.
101. Hirigoyen MB, Blackwell KE, Zhang WX, Silver L, Weinberg H, Urken ML. Continuous tissue oxygen tension measurement as a monitor of free-flap viability. Plast Reconstr Surg 1997;99:763-73.
102. Gosain A, Rabkin J, Reymond JP, Jensen JA, Hunt TK, Upton RA. Tissue oxygen tension and other indicators of blood loss or organ perfusion during graded hemorrhage. Surgery 1991;109:523-32.
103. Gottrup F, Firmin R, Chang N, Goodson WH 3rd, Hunt TK. Continuous direct tissue oxygen tension measurement by a new method using an implantable silastic tonometer and oxygen polarography. Am J Surg 1983;146:399-403.
104. Trignano E, Fallico N, Fiorot L, et al. Flap monitoring with continuous oxygen partial tension measurement in breast reconstructive surgery: a preliminary report. Microsurgery 2018;38:402-6.
105. Clark LC Jr. Measurement of oxygen tension: a historical perspective. Crit Care Med 1981;9:690-2.
106. Liss AG, Liss P. Use of a modified oxygen microelectrode and laser-doppler flowmetry to monitor changes in oxygen tension and microcirculation in a flap. Plast Reconstr Surg 2000;105:2072-8.
107. Hunt TK, Rabkin J, Jensen JA, Jonsson K, von Smitten K, Goodson WH 3rd. Tissue oximetry: an interim report. World J Surg 1987;11:126-32.
108. Chang N, Goodson WH 3rd, Gottrup F, Hunt TK. Direct measurement of wound and tissue oxygen tension in postoperative patients. Ann Surg 1983;197:470-8.
109. Gottrup F, Firmin R, Hunt TK, Mathes SJ. The dynamic properties of tissue oxygen in healing flaps. Surgery 1984;95:527-36.
110. Schrey A, Niemi T, Kinnunen I, et al. The limitations of tissue-oxygen measurement and positron emission tomography as additional methods for postoperative breast reconstruction free-flap monitoring. J Plast Reconstr Aesthet Surg 2010;63:314-21.
111. Mccraw JB, Myers B, Shanklin KD. The value of fluorescein in predicting the viability of arterialized flaps. Plast Reconstr Surg 1977;60:710-9.
112. Adelsberger R, Fakin R, Mirtschink S, Forster N, Giovanoli P, Lindenblatt N. Bedside monitoring of free flaps using ICG-fluorescence angiography significantly improves detection of postoperative perfusion impairment#. J Plast Surg Hand Surg 2019;53:149-54.
113. Nagata T, Masumoto K, Uchiyama Y, et al. Improved technique for evaluating oral free flaps by pinprick testing assisted by indocyanine green near-infrared fluorescence angiography. J Craniomaxillofac Surg 2014;42:1112-6.
114. Hitier M, Cracowski JL, Hamou C, Righini C, Bettega G. Indocyanine green fluorescence angiography for free flap monitoring: a pilot study. J Craniomaxillofac Surg 2016;44:1833-41.
115. Su CT, Im MJ, Hoopes JE. Tissue glucose and lactate following vascular occlusion in island skin flaps. Plast Reconstr Surg 1982;70:202-5.
116. Bashir MM, Tayyab Z, Afzal S, Khan FA. Diagnostic accuracy of blood glucose measurements in detecting venous compromise in flaps. J Craniofac Surg 2015;26:1492-4.
117. Karakawa R, Yoshimatsu H, Narushima M, Iida T. Ratio of blood glucose level change measurement for flap monitoring. Plast Reconstr Surg Glob Open 2018;6:e1851.
118. Dickson MG, Sharpe DT. Continuous subcutaneous tissue pH measurement as a monitor of blood flow in skin flaps: an experimental study. Br J Plast Surg 1985;38:39-42.
119. Sakakibara S, Hashikawa K, Omori M, Terashi H, Tahara S. A simplest method of flap monitoring. J Reconstr Microsurg 2010;26:433-4.
120. Hara H, Mihara M, Iida T, et al. Blood glucose measurement for flap monitoring to salvage flaps from venous thrombosis. J Plast Reconstr Aesthet Surg 2012;65:616-9.
121. Henault B, Pluvy I, Pauchot J, Sinna R, Labruère-Chazal C, Zwetyenga N. Capillary measurement of lactate and glucose for free flap monitoring. Ann Chir Plast Esthet 2014;59:15-21.
122. Sitzman TJ, Hanson SE, King TW, Gutowski KA. Detection of flap venous and arterial occlusion using interstitial glucose monitoring in a rodent model. Plast Reconstr Surg 2010;126:71-9.
123. Zhang C, Wang Q, Wu L, Wang J, Zhao S, Wang J. Continuous interstitial glucose measurement for flap venous occlusion monitoring in a diabetic model. J Craniofac Surg 2022;33:2698-703.
124. Giatsidis G. Discussion: flap blood glucose as a sensitive and specific indicator for flap venous congestion: a rodent model study. Plast Reconstr Surg 2019;144:419e-20e.
125. Mochizuki K, Mochizuki M, Gonda K. Flap blood glucose as a sensitive and specific indicator for flap venous congestion: a rodent model study. Plast Reconstr Surg 2019;144:409e-18e.
126. Sommer T. Microdialysis of the bowel: the possibility of monitoring intestinal ischemia. Expert Rev Med Devices 2005;2:277-86.
127. Dakpé S, Colin E, Bettoni J, et al. Intraosseous microdialysis for bone free flap monitoring in head and neck reconstructive surgery: a prospective pilot study. Microsurgery 2020;40:315-23.
128. Udesen A, Løntoft E, Kristensen SR. Monitoring of free TRAM flaps with microdialysis. J Reconstr Microsurg 2000;16:101-6.
129. Röjdmark J, Blomqvist L, Malm M, Adams-Ray B, Ungerstedt U. Metabolism in myocutaneous flaps studied by in situ microdialysis. Scand J Plast Reconstr Surg Hand Surg 1998;32:27-34.
130. Jyränki J, Suominen S, Vuola J, Bäck L. Microdialysis in clinical practice: monitoring intraoral free flaps. Ann Plast Surg 2006;56:387-93.
131. Top H, Sarikaya A, Aygit AC, Benlier E, Kiyak M. Review of monitoring free muscle flap transfers in reconstructive surgery: role of 99mTc sestamibi scintigraphy. Nucl Med Commun 2006;27:91-8.
132. Aygit AC, Sarikaya A. Technetium 99m sestamibi scintigraphy for noninvasive assessment of muscle flap viability. Ann Plas Surg 1999;43:338-40.
133. Sarikaya A, Aygit AC. Combined 99mTc MDP bone SPECT and 99mTc sestamibi muscle SPECT for assessment of bone regrowth and free muscle flap viability in an electrical burn of scalp. Burns 2003;29:385-8.
134. Prantl L, Fellner C, Jung ME. Evaluation of free flap perfusion with dynamic contrast-enhanced magnetic resonance imaging. Plast Reconstr Surg 2010;126:100e-1e.
135. Fellner C, Jung EM, Prantl L. Dynamic contrast-enhanced MRI as a valuable non-invasive tool to evaluate tissue perfusion of free flaps: preliminary results. Clin Hemorheol Microcirc 2010;46:77-87.
136. Lee C, Chen C, Wang H, Chen L, Perng C. Utilizing mask RCNN for monitoring postoperative free flap: circulatory compromise detection based on visible-light and infrared images. IEEE Access 2022;10:109510-25.
137. Lee C, Chen C, Hsu F, et al. A postoperative free flap monitoring system: circulatory compromise detection based on visible-light image. IEEE Access 2022;10:4649-65.
138. Matsui C, Lao WW, Tanaka T, et al. Real-time assessment of free flap capillary circulation using videocapillaroscopy. Plast Reconstr Surg 2022;150:407-13.
139. Bucknor A, Kamali P, Maylar M, et al. Abstract 126: Improving post-operative monitoring of autologous breast reconstruction: a novel, oxygen-sensing liquid bandage first-in-human trial. Prs-Glob Open 2018;6:98-9.
Cite This Article
Export citation file: BibTeX | RIS
OAE Style
Cevallos PC, Najafali D, Johnstone TM, Borrelli MR, Manrique OJ, Lee GK, Nazerali RS. Detecting flap compromise: an updated review of techniques to monitor microsurgical flaps post-operatively in breast reconstruction. Plast Aesthet Res 2023;10:30. http://dx.doi.org/10.20517/2347-9264.2023.01
AMA Style
Cevallos PC, Najafali D, Johnstone TM, Borrelli MR, Manrique OJ, Lee GK, Nazerali RS. Detecting flap compromise: an updated review of techniques to monitor microsurgical flaps post-operatively in breast reconstruction. Plastic and Aesthetic Research. 2023; 10: 30. http://dx.doi.org/10.20517/2347-9264.2023.01
Chicago/Turabian Style
Cevallos, Priscila C., Daniel Najafali, Thomas M. Johnstone, Mimi R. Borrelli, Oscar J. Manrique, Gordon K. Lee, Rahim S. Nazerali. 2023. "Detecting flap compromise: an updated review of techniques to monitor microsurgical flaps post-operatively in breast reconstruction" Plastic and Aesthetic Research. 10: 30. http://dx.doi.org/10.20517/2347-9264.2023.01
ACS Style
Cevallos, PC.; Najafali D.; Johnstone TM.; Borrelli MR.; Manrique OJ.; Lee GK.; Nazerali RS. Detecting flap compromise: an updated review of techniques to monitor microsurgical flaps post-operatively in breast reconstruction. Plast. Aesthet. Res. 2023, 10, 30. http://dx.doi.org/10.20517/2347-9264.2023.01
About This Article
Special Issue
Copyright
Data & Comments
Data
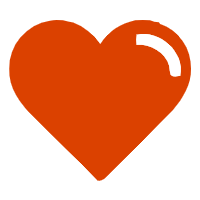

Comments
Comments must be written in English. Spam, offensive content, impersonation, and private information will not be permitted. If any comment is reported and identified as inappropriate content by OAE staff, the comment will be removed without notice. If you have any queries or need any help, please contact us at support@oaepublish.com.